NCBI Bookshelf. A service of the National Library of Medicine, National Institutes of Health.
Madame Curie Bioscience Database [Internet]. Austin (TX): Landes Bioscience; 2000-2013.
Cell death following cerebral ischemia is mediated by a complex pathophysiologic interaction of different mechanisms. In this Chapter we will outline the basic principles as well as introduce in vitro and in vivo models of cerebral ischemia. Mechanistically, excitotoxicity, peri-infarct depolarization, inflammation and apoptosis seem to be the most relevant mediators of damage and are promising targets for neuroprotective strategies.
Epidemiological Data
The incidence of stroke in the Federal Republic of Germany (a population of 81 million people) is approximately 250-400/100,000. Hence, an estimated total of 200,000 strokes occur per year. Stroke mortality amounts—irrespective of all therapeutic efforts—still are 25 to 30%. Stroke is the third leading cause of death in the industrialized world. For example, in Germany there are two million stroke victims alive (for comparison, four million in the United States of America). Stroke is the leading cause of disability; its direct and indirect costs amount to approximately 30 to 40 billion US$ per year.
Introduction
The metabolism of the brain depends exclusively on oxygen and glucose and adds up to the consumption of 75 l molecular oxygen and 120 g glucose per day. In comparison to other organs this situation is unique. Albeit the weight of the brain accounts for only 2% of the total body weight, the brain claims 20% of total body perfusion and 20% of total oxygen consumption. Even short durations of reduced brain perfusion leading to lack of oxygen and energy metabolites may lead to irreversible structural damage. Variable “ischemia thresholds” that are region- and cell type-specific determine whether a respective cell will survive the insult or die (see also Table 1).
Table 1
Perfusion thresholds during cerebral ischemia.
Global vs. Focal Ischemia
In general, there are two mechanistically distinct modes of cerebral ischemia, i.e., global and focal ischemia, respectively. Global ischemia in man develops after transient circulatory arrest with resuscitation or after near-drowning (Table 2). Consequently, absolute cerebral blood flow falls off from 0.8 ml/g/min to zero within seconds. Loss of consciousness follows after approximately 10 seconds. EEG activity ceases after 30 to 40 secs, and a few minutes of global ischemia lead to irreversible cellular damage that evolves over days. The typical histological picture following global ischemic insults is described by delayed neuronal death sparing glial cells (sometimes even associated with astrogliosis). As a general rule, under normothermic conditions, 10 min of global ischemia are lethal in man. In the United States approximately 500,000 people/year die because of circulatory arrest leading to global ischemia.
Focal ischemia follows transient or permanent flow reduction in the territory of a cerebral artery. Typically, flow reduction is due to embolic or thrombotic vessel occlusion. In contrast to the situation after global ischemia, focal ischemia is characterized by the formation of a so-called “ischemic penumbra”. The penumbra is defined as the ischemia border-zone which is (still) metabolically active but functionally silent. While absolute regional blood flow in the ischemic core is diminished to levels <0.1 ml/g/min, blood flow in the penumbra typically remains at 0.2-0.4 ml/g/min. The typical histological picture following focal ischemia is a pan-necrosis that includes all cell types in the brain (neurons, astrocytes, oligodendrocytes, endothelial cells).
Animal Models of Cerebral Ischemia
Although stroke has been studied in many species (for example rabbits, dogs, cats, and baboons), rats and mice are the most widely investigated.1 Mice are especially useful because of the availability of unique strains that can be genetically engineered to over- or underexpress targeted genes.2-4 Several well-established models are available to study global ischemia. In the so-called “four-vessel occlusion model” (4VO), flow in both carotid arteries and vertebral arteries is blocked for a specified time period (“Pulsinelli-Brierley”-model).6 In the two-vessel occlusion model (2VO) which is also referred to as “severe forebrain ischemia”, only the carotid arteries are temporarily occluded, sometimes along with mild hypotension.7 In these models, injury develops selectively in cells most vulnerable to ischemic damage such as in the CA1 sector in hippocampus, medium-sized neurons in the striatum, and Purkinje cells in cerebellum. Neurons are more susceptible than glial cells, and die over hours to days after the insult; hence the term “delayed neuronal death”. Experimental focal ischemia is most commonly studied during permanent or transient occlusion of a middle cerebral artery (MCA).8 - 10 Proximal MCA occlusion can be induced by an intraluminal suture (so-called filament model) or with a vascular clip and causes injury to cortex and deep structures (striatum). Distal MCA occlusion (the so-called “Brint-model”) is usually produced by placing a vascular clip on a pial vessel or by cautery.11 The occlusion typically spares striatum and primarily involves the neocortex. Pan-necrosis develops in the territory supplied by the respective artery with glial and endothelial cell death. If recirculation is established early (2 hrs or less) outcome is better (transient MCA occlusion).12 In some ways, the reperfused brain imitates restoration of blood flow after spontaneous lysis of a thrombo-embolic clot in humans, even though reperfusion after clot lysis is certainly more complex than an on/off phenomenon as modelled by placement and retraction of an intravascular filament. During reperfusion, free radical production and NO generation are especially pronounced and contribute to “reperfusion injury”.13 After longer times of ischemia, reperfusion is incomplete due to microvascular occlusion which has been termed the “no-reflow phenomenon” some thirty years ago.14 Because oxygen-free radicals and NO promote apoptotic cell death, transient ischemia models have become especially useful to investigate cell death in vivo15,16 which may particularly apply for models of “mild ischemia”.17-19 In these models apoptosis is prominent after 30 min MCA occlusion followed by longer reperfusion times (several days).19,20 The pattern of cell death is reminiscent of global ischemia in that it is both selective for neurons and delayed. Mild ischemia models may be similar to transient ischemic attacks in man. In fact, changes in T1/T2-weighted MR imaging 7 days after 15 min MCAo occlusion in rats resemble those 7 to 10 days after transient ischemic attacks (TIA's) in patients with known cardiogenic embolism. However, selective neuronal death has not yet been convincingly documented following TIA's in humans.
In Vitro Models of Cerebral Ischemia
To study the effects of “cerebral ischemia” in post-mitotic neurons in vitro, the so-called “oxygen-glucose deprivation” (OGD) model is commonly used. Primary cultures of post-mitotic neurons from different regions of the brain (such as cortex, striatum, septum, hippocampus, etc.) can be established from rat or mouse embryos (day 16 to 18). After several days in vitro (10 to 14 days) these post-mitotic cells can be exposed to a combined deprivation of oxygen and glucose. Depending on the length and severity of the insult cell death develops and can be quantified on a morphological, biochemical, or molecular basis.21,22 Other possibilities to study ischemic damage in vitro include the studies of brain slices, particularly the hippocampal slice. Usually, the bathing solution is changed from a mixture of oxygen/carbon dioxide to nitrogen/carbon dioxide in the absence (hence “ischemia”) or presence (hence “anoxia”) of glucose. Generally, 5-7 min of ischemia lead to profound cell loss in the CA1 region. Shorter insults lead to a more slowly evolving damage, requiring approximately 12 h to be manifested. This type of cell death in many ways resembles “delayed neuronal death” seen in vivo.
Importance of Physiologic Parameters for Stroke Outcome
Both animal and clinical studies have proven that changes in physiologic parameters early after stroke onset influence ischemia outcome. Of great importance are changes in brain and body temperature, partial pressures of oxygen and carbon dioxide, mean arterial blood pressure and glucose metabolism which may have direct impact on the treatment of stroke patients.
Temperature: Fever is a frequent complication following stroke, and increases in body and brain temperature are associated with poor stroke outcome. Experimental studies unequivocally demonstrate that hypothermia reduces lesion volume while hyperthermia increases cell death following global ischemia (Table 3) and lesion volume after focal ischemia. Reducing temperature to 30°C for 1 h after 2 h transient occlusion of the middle cerebral artery reduced lesion volume by 50%. This has direct implications for the treatment of acute stroke victims. Even slight elevations of body temperature (>37.5°C) should be normalized by physical and pharmacological means. Current studies investigate acute treatment protocols using hypothermia.
Table 3
Effects of temperature on damage following global cerebral ischemia.
Oxygen and carbon dioxide: Both hypoxia as well as hypercapnia have an adverse effect on stroke outcome in animal models of cerebral ischemia. Theoretically, hypercapnia has advantageous effects by inducing vasodilation. Perfusion in the penumbra, however, is decreased during hypercapnia possibly due to a “steal” phenomenon.
Mean arterial blood pressure: Cerebral autoregulation is severely impeded during cerebral ischemia. Hence, brain perfusion is directly dependent on mean arterial blood pressure. Recent experimental studies have indeed demonstrated that blood pressure lowering increases infarct size in spontaneously hypertensive rats.23 As a consequence, blood pressure in patients should be maintained at relatively high levels to improve brain perfusion and thus outcome.
Glucose: Albeit loss of glucose is one of the initiators of cell death after cerebral ischemia, elevated levels of blood glucose are nevertheless deleterious following focal ischemia. Accumulation of lactate by anaerobic glycolysis leads to acidosis with adverse effects on ischemic tissue.
Pathophysiological Cascades Following Cerebral Ischemia
Loss of oxygen and energy depletion initiates a self-promoting cascade of pathophysiologic events that evolve over minutes, hours, days, and even weeks. In this Chapter we will follow a putative sequence of events that can be differentiated in (1) excitotoxicity, (2) peri-infarct depolarization, (3) inflammation, and (4) apoptosis. Obviously, this is an oversimplification and both mechanistically and in terms of temporal evolution there is substantial overlap.24
Excitotoxicity and Energy Depletion
As mentioned above brain tissue has an high energy demand. Moreover, to generate energy metabolites the brain is exclusively dependent on oxidative phosphorylation. Following focal ischemia there is profound loss of both oxygen and glucose. Within minutes energy-dependent ion channels become activated which leads to the loss of the membrane potential.25 Both neuronal and non-neuronal cells become depolarized and voltage-dependent Ca-channels are activated. Depolarization also induces release of neurotransmitters such as excitatory amino acids (glutamate) from presynaptic axon terminals into the synaptic cleft.26 Since re-uptake mechanisms have failed, these excitatory amino acids subsequently accumulate in the synaptic cleft and induce activation of ligand-gated Ca-permeable channels (such as of the NMDA and AMPA subtype) as well as metabotropic Ca-channels.
Subsequently, there is influx of other extracellular ions (Na, Cl) that accumulate intracellulary and lead to cell swelling and intracellular (“cytotoxic”) edema. This intracellular edema can be visualized by magnetic resonance tomography using diffusion weighted imaging (DWI) techniques, providing the most sensitive non-invasive modality for detecting ischemia-induced tissue injury. Using perfusion weighted imaging (PWI), it is possible to compare regions of compromised blood flow (PWI) with already lesioned tissue (DWI). The difference between both volumes (“mismatch”) might correspond to salvageable “penumbra” tissue. The latter hypothesis is currently being tested in a number of clinical trials.
The most important trigger for all subsequent events leading to cellular disruption and cell death is the intracellular increase of calcium ions: Ca acts as a universal second and third messenger and triggers via enzyme induction multiple cytoplasmic and nuclear cascades: Ca activates a number of important enzymes: (1) proteolytic enzymes (such as calpain, gelsolin etc.) with subsequent degradation of the cytoskeleton and structural proteins (e.g., actin, laminin, spectrin, microtubuli-associated proteins);27-29 (2) xanthine oxidase and phospholipases (such as phospholipase A2) which leads to membrane degradation, (3) cyclooxygenase leading to free radical generation, (4) neuronal type nitric oxide synthase (nNOS) leading to the production of nitric oxide (NO) and NO-derived radical peroxynitrite by the reaction with superoxide.30,31
Nitric oxide plays a critical role during cerebral ischemia. Importantly, NO may exert beneficial as well a deleterious effects depending on the time-point and compartment of its production. Hence, analysis of the role of NO during cerebral ischemia has led to much confusion in the literature and was described as a “double-edged sword”. NO is synthetized from L-arginine and oxygen by NO synthases (NOS). There are two constitutive isoforms, neuronal (type I) and endothelial (type III), which are calcium/calmodulin-dependent, and one inducible isoform (iNOS, type II). Small quanta of NO synthetized by constitutive NOS regulate a wide variety of physiological functions such as blood pressure, vascular tone, permeability and neurotransmission.32 iNOS can be induced in microglia, astrocytes, endothelium, and vascular smooth muscle. Once expressed, it is continuously active, irrespective of intracellular calcium levels and leads to high output NO synthesis leading to cytotoxicity and inflammatory actions. Like iNOS, nNOS can also generate high amounts of NO and cause cytotoxicity under pathophysiological conditions, due to the above mentioned intracellular rise of Ca.21 Although nNOS-positive neurons comprise only 1–2% of all neurons they possess extensive branching. Of note, the nNOS-positive neurons themselves are surprisingly resistant against injury. Cortical levels of NO increase strikingly from approximately 10 nM to 2 μM within a few minutes after MCA occlusion. Animals lacking nNOS expression (nNOS knockout animals) have 38% smaller cerebral infarcts following permanent MCAo than control mice, unequivocally demonstrating the deleterious role of nNOS-derived NO during cerebral ischemia. NO neurotoxicity is mediated most likely by peroxynitrite formed by the reaction of NO with superoxide, a complex that rapidly decomposes into hydroxyl radicals, which are a highly reactive species.
An attractive downstream candidate for mediating NO-induced neurotoxicity is activation of the nuclear enyzme poly(ADP-ribose)polymerase (PARP). Formation of single-stranded DNA nicks (for example by peroxynitrite) is an obligatory stimulus of PARP leading to the formation of poly-ADP-ribose chains and depletion of its intracellular substrate NAD.33,34 PARP is activated within minutes following cerebral ischemia and reperfusion35 and inhibition of PARP activation or deletion of the PARP gene confers protection after ischemia.35,36 Consistent with the notion that PARP is a perpetrator in NO-mediated neurotoxicity nNOS knockout animals had strikingly reduced levels of PARP activation within ischemic tissue.37
On the other hand, NO produced by the endothelial NOS isoform may exert protective effects via augmentation of cerebral blood flow, inhibition of platelet aggregation and leukocyte activation. Animals lacking expression of the endothelial NOS subtype (Type III) have been generated (eNOS knockout mice ).38 Expectedly, these animals have elevated arterial blood pressure. Moreover, eNOS knockout animals develop enlarged cerebral infarcts following middle cerebral artery occlusion, demonstrating a protective role of type III NOS during cerebral ischemia. The susceptibility of eNOS mutants to ischemic injury may be due to their diminished capacity to adapt to reduced perfusion pressure (i.e.dilate) at the margins of an ischemic lesion. This coupled to enhanced platelet and neutrophil adhesion, renders eNOS mutants susceptible to injury. Consistent with this notion, blocking NOS activity by nitro-L-arginine administration increased infarct size in nNOS knockout animals, presumably due to inhibition of the constitutively expressed eNOS isoform.39In conclusion, genetic evidence suggests that two NOS isoforms, i.e., nNOS and iNOS, contribute to ischemic injury, most likely due to the generation of NO-derived radicals, while NO generated by another NOS isoform, i.e., eNOS, is protective by its effect on blood flow and platelet aggregation. Pharmacologic approaches for acute treatment should be directed at selective inhibition of nNOS and iNOS isoforms while eNOS activity should be augmented. Recently, statins (HMG-CoA reductase inhibitors) were shown to selectively upregulate eNOS activity and NO production after chronic administration. Mice treated for 14 days with statins had increased eNOS message and activity, augmented cerebral blood flow and decreased markers of platelet activation, and had significantly smaller stroke sizes after MCAo.40,41
Another important source for the generation of reactive oxygen species (ROS) are mitochondria, which may themselves be damaged by ROS initiating a vicious cycle.42,43 Specifically, the inner mitochondrial membrane is severely damaged by the generation of radicals and also by formation of a so-called “mitochondrial permeability transition pore” (MPT), which leads to organ swelling, generation of additional ROS and finally cessation of ATP production. This may be coupled to the specific or unspecific release of cytochrome c into the cytosol which has been recognized as a specific upstream trigger for the initiation of the proteolytic caspase cascade leading to apoptotic cell death (Fig. 1).44
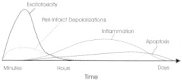
Figure 1
Putative cascade of damaging events in focal cerebral ischemia.
Hemodynamic, metabolic, and ionic phenomena are not homogeneous in different ischemic brain regions. In the center or core of the ischemic region, cerebral blood flow is less than 20% of normal levels. In this region, permanent and anoxic depolarizations develop minutes after onset of ischemia. Subsequently, cells are killed rapidly by lipolysis, proteolysis and disruption of the cytoskeleton leading to loss of energy and ion homeostasis.45 Between this core region and normal, non-ischemic tissue lies the so-called penumbra, an ischemic border-zone which is functionally silent but metabolically (still) active. In theory, the whole penumbra region can be salvaged by timely therapeutic intervention. Over time, however, and without treatment the “core grows on the cost of the penumbra”, i.e., the penumbra can progress to infarction by mechanisms described in this Chapter.46-48Hence, the goal of all therapeutic interventions is to protect the ischemic penumbra region. Albeit there is good evidence for the existence of a penumbra during human stroke,49,50 its size and pathophysiologic relevance remains unclear.51
Glutamate Receptors and Excitotoxicity
Activation of glutamate receptors and subsequent calcium influx leading to increased intra-cellular calcium levels ([Ca]i) may be the most important early trigger of subsequent cell death after cerebral ischemia. Consequently, pharmacological inhibition of these receptors is an attractive treatment approach for stroke. Three different ligand-gated glutamate receptors can be differentiated based on their respective pharmacological affinities:(1) N-methyl-D-aspartate (NMDA) receptor, (2) α-amino-3 hydroxy-5 methyl-4- isoxazolone-proprionic acid (AMPA) receptor, and (3) kainate receptor. In addition, there are metabotropic G-protein coupled receptors.
NMDA receptors are permeable for Ca, Na and K ions. Following NMDA-receptor activation three stages inducing neurotoxicity can be differentiated: (1) changes in the intracellular milieu (induction), (2) exponential increase in [Ca]i (amplification), (3) neuronal degeneration (expression). There are several pharmacologically distinct receptor antagonists available: NMDA receptor antagonists (such as MK-801) inhibit almost completely intracellular Ca-influx in primary neurons following “oxygen-glucose-deprivation” in vitro. NMDA receptor blockage using competitive and non-competitive receptor antagonists confers robust neuroprotection in animal model of focal (but not global) cerebral ischemia. Administration of NMDA receptor antagonists is neuroprotective only within a very limited “window of opportunity” (minutes to a few hours), however. Moreover, severe adverse effects of NMDA receptor blocker were demonstrated including exogenous psychosis and specific cell loss in the limbic system.52,53 Currently, site-specific antagonists (for example for the glycine site) are being tested as a possible alternative.
AMPA receptors mediate Na and K currents. Na-influx via AMPA as well as kainate receptors leads in turn to secondary influx of Ca-ions. Pharmacological blockade of these receptors via specific inhibitors confers significant reduction of infarct volume in animal stroke models. Of note, the treatment window is somewhat better than with NMDA receptor antagonists.54 In contrast to the above listed ligand-gated glutamate receptors, the role of metabotropic glutamate receptors during cerebral ischemia has so far remained unclear. Some studies suggest a protective role for some classes of metabotropic receptors.55
Tissue Acidosis
Following oxygen deprivation glucose metabolism is changed to anaerobic glycolysis leading to lactate accumulation and tissue acidosis. For a long time acidosis was recognized as a central pathomechanism of tissue injury following stroke. For example, neurons in culture die rapidly when exposed to low pH. This has been explained by increased radical production and other mechanisms at low pH which may mediate damage. Recent observations, however, have shed new light on the dogma that acidosis is always dismal after cerebral ischemia. For example, NMDA receptors become inactivated at low pH levels protecting neurons from further Ca-influx.
Protein Synthesis and Early Gene Expression
Even slight reductions of regional cerebral blood flow compromise total protein synthesis. In spite of these inhibitory effects on general protein synthesis there are some genes and gene products that are upregulated both on a transcriptional and translational level. Among these are the so-called “immediate early genes” whose gene products act as transcription factors. Via expression of these early genes both protective and destructive cascades are initiated.
Peri-infarct Depolarization
Loss of energy and glutamate release induce depolarization of neurons and non-neuronal cells. In the core of the ischemic lesion this may induce so-called anoxic depolarization: Due to lack of energy equivalent the cells are unable to repolarize and are destined to die. In the border-zone (“penumbra”) of the lesion, however, cells are able to re-polarize, but at the expense of further energy depletion. Waves of de- and re-polarization may establish which are called peri-infarct depolarizations.56, 57 Evidence of this phenomenon has been provided in several animal models and a number of observations argue that it may have pathophysiological relevance. (1) Peri-infarct depolarizations (PIDs) may occur several times per hour and can be detected up to 6-8 hours after ischemia onset. (2) Lesion volume and cell loss correspond to the number of PIDs.58 (3) Reduction of PIDs by therapeutic intervention reduces infarct volume.59 (4) Induction of additional PIDs by topical KCl administration further increases lesion volume. Albeit experimental evidence for PIDs as a relevant pathomechanism after stroke is convincing, so far evidence for the occurrence of PIDs after stroke in man is lacking.60
Inflammation
Expression of proinflammatory genes, such as NF-κB, hypoxia-inducible factor and interferon 1b is triggered early after onset of ischemia.61-63 These proinflammatory genes may initiate the production of several mediators of inflammation including platelet activation factor and tumor necrosis factor. In turn, these factors themselves initiate a cascade of events that includes the expression of adhesion molecules of endothelial cells (intercellular and vascular adhesion molecules; ICAM and VCAM as well as different selectins). Adhesion molecules mediate rolling, adhesion (“sticking”) and finally migration of leukocytes through the vascular wall.64-66 In a first wave neutrophils invade the brain which is followed by macrophages and monocytes. The latter express typical chemokines (e.g., interleukin-8 and monocyte chemoattractant protein-1) leading to a vicious cycle.
The x-axis reflects the evolution of the cascade over time, while the y-axis illustrates the impact of each element of the cascade on final outcome (adapted from Dirnagl et al, TINS 22:391-397). However, not only blood-born cells mediate inflammatory responses after ischemia but also brain-derived immunocompetent cells such as microglia. Microglial cells constitute the primary immunoeffector cells of the brain and amount to up to 20% of total brain cell number. Within hours after onset of an insult these cells become activated microglia. The question, however, whether inflammation after cerebral ischemia is “good or bad” has not unequivocally been resolved although the literature is in favor of a destructive effect. For example, (1) induction of neutropenia, (2) inhibition of several inflammatory mediators (such as Il-1b) or (3) adhesion molecules significantly protects from cell death and improves outcome after stroke.67,68
There are a plethora of molecular and biological mechanisms that contribute to inflammation-mediated cellular damage: The cerebral microcirculation becomes severely compromised by leukocyte plugging of small vessels. Neurons and macrophages may induce toxic enzymes such as inducible NO synthase (iNOS) or cyclooxygenase (COX). iNOS is produced by invading neutrophils which may lead to increased NO production. With the use of pharmacological inhibition or genetic deletion (iNOS knockout mouse) it has been unequivocally demonstrated that iNOS exerts neurotoxic effects during cerebral ischemia. Of note, the pathomechanism of iNOS induced cytotoxicity is a delayed one: iNOS becomes expressed only 24 h after the onset of the insult and administration of iNOS inhibitors is neuroprotective even when administered one day after stroke onset.63,69,70 COX-2 on the other hand is produced by neurons. It amplifies ischemic damage by production of superoxide anions and toxic prostanoids.71 The role of yet another inflammatory mediator, TNF-alpha, during cerebral ischemia has so far remained controversial.72,73
Apoptosis
The term “apoptosis” was first introduced by Kerr et al in 1972.74 Hallmarks of apoptosis include chromatin condensation, nuclear segmentation, cytoplasmic shrinkage, blebbing, and formation of apoptotic bodies (See Table 4). The possibility that ischemic brain cells may also die by apoptotic mechanisms was not proposed until 1993.75-77 In fact, ischemic cell death was considered the classical prototype for necrotic cell death based mainly on morphological criteria. Necrosis describes swollen cells with disruption of subcellular organelles and is also called “accidental cell death”.78 (see also Table 4). Now convincing biochemical, histochemical, molecular and genetic data favor apoptosis (or a closely related mechanism) as an additional mechanism of cell death in models of cerebral ischemia. There are numerous reports demonstrating oligonucleosomal DNA fragmentation either by gel electrophoresis (“DNA laddering”) or by the TUNEL in situ technique (“terminal deoxynucleotidyl transferase mediated dUTP-biotin nick-end labeling”).75,77,79-82 Following focal ischemia apoptosis is predominant in the ischemic border-zone.75,83,84Following more severe insults TUNEL-positive cells appear earlier than after mild insults.19 DNA laddering and TUNEL staining, however, are not pathognomonic for apoptosis. Most neuropathologists accept electron microscopy criteria as the gold standard. Van Lookeren Campagne and Gill85 found 1996 no ultrastructural evidence for apoptosis after MCA occlusion in the rat.
Table 4
Apoptosis vs. necrosis.
It is generally accepted that new protein synthesis is needed for the initiation of apoptosis.74 In fact, the protein synthesis inhibitor cycloheximide protects cells and reduces injury after focal ischemia.18,19,75 In general, cycloheximide suppresses synthesis of all proteins, including those that protect cells. Hence, is has remained elusive by inhibition of which downstream products neuroprotection of cycloheximide is mediated.
Molecular Mechanisms
During development, apoptosis is triggered by evolutionally highly conserved signals, and is regulated by a balance between death promoting and death inhibiting factors.86-89 In the nematode Caenorhabditis elegans the death promoting ced-3 and ced-4 genes and the death inhibiting gene ced-9 were identified.90-93 As mammalian homologues for the ced-3 gene the so-called caspases (c: cystein proteinase; -aspase: cleavage after aspartate residues) were identified (formerly also known as interleukin-1β family).94,95 These proteases consist of at least 12 familiy members and are important executioners of apoptosis. The most important caspase for apoptosis execution is caspase-3. Caspase-3, as all caspases, is activated by proteolytic cleavage (posttranslational modification) and in turn cleaves itself multiple (>30) substrates. Known caspase-3 substrates are for example endonuclease (CAD plus inhibitor ICAD), lamin, spectrin, huntingtin, gelsolin, poly(ADP-ribose)polymerase (PARP, see above). Cleavage of these caspase substrates is thought to mediate the downstream events during apoptosis (beyond the point of “no-return”). Until recently, it had remained unclear how caspase-3 is activated during cerebral ischemia. Generally, two pathways exist: type I apoptosis via Fas/TNF-receptors, activation of intracellular death recepors and activation of caspase-8; type II apoptosis via release of cytochrome c from mitochondria, formation of the so-called “apoptosome” (which is a complex of cytochrome c, apoptosis activation factor and pro-caspase-9).96 Hence, after their respective cleavage either caspase-8 (type I) or caspase-9 (type II) activate caspase-3. Recent data favours the idea that caspase-3 is activated during cerebral ischemia predominantly via release of cytochrome c into the cytosol.28
Experimental Evidence for Caspase-Mediated Cell Death Following Cerebral Ischemia
Both caspase-1 and -3 become activated in the ischemic territory following focal cerebral ischemia,19,97an event that precedes markers of morphological damage and TUNEL staining by hours. Animals with deletion of caspase-1 or caspase-3 have smaller ischemic lesions.98-100Following a general rule that severe insults lead to necrotic cell death while after milder insults apoptosis is prominent, caspase activation was analyzed following short durations of MCA occlusion. Following 30 min MCAo caspase-3 was not activated until 9-12 hours after ischemia onset, while after 2 h MCAo it was active within minutes after reperfusion. More than 50% of all neurons within the ischemic tissue double stain for activated caspase-3 and TUNEL.20,97
Caspase Inhibition Protects From Cerebral Ischemia
Small oligopeptides (tri- or tetrapeptides) that mimic the cleavage site of a protease substrate can be used as relatively selective caspase inhibitors.101,102For example YVAD.cmk (acetyl-Tyr-Val-Ala-Asp.chloromethylketone) is relatively selective for caspase-1 (Ki 0.76 nM) while zDEVD.fmk (N-benzyloxycarbonal.Asp-Glu-Val-Asp-fluoromethylketone) is more selective for caspase-3 (Ki <0.1 nM).103,104 Intracerebroventricular administration of these inhibitors blocks the activation of capase-1 and -3, respectively, and significantly protects from injury following middle cerebral artery occlusion and global ischemia.19,20,100,105,106Following mild insults the treatment window was extended to 9 hr after the onset of ischemia.19,20Interestingly, caspase inhibitors act synergistically with anti-excitotoxic drugs such as the glutamate receptor blocker MK-801.107 Hence, it seems feasible that both anti-excitotoxic and anti-apoptotic drugs may be given in a “neuroprotective cocktail”; low doses may limit potential toxicity from either drug alone. Of note, non-peptide caspase inhibitors are being developed that can be administered systemically.
Conclusion
Cell death following cerebral ischemia is mediated by a complex pathophysiologic interaction of different mechanisms. As outlined in this Chapter, excitotoxicity, peri-infarct depolarization, inflammation, and apoptosis seem to be the most relevant mediators and are promising targets for neuroprotective intervention. After all, however, stroke is a vascular disease. Reperfusion of an occluded artery (e.g., by rt-PA) is still the most important early intervention and should be combined with neuroprotective strategies.
References
- 1.
- Ginsberg MD. Animal models of global and focal cerebral ischemia In: Welsh KMA, Caplan LC, Reis DJ, Siesjo BK, Weir B, eds. Primer on Cerebrovascular Disease San Diego: Academic Press, 1997124–126.
- 2.
- Fässler R, Martin K, Forsberg E. et al. Knockout mice: how to make them and why. The immonological approach. Int Arch Allergy Immunol. 1995;106:323–334. [PubMed: 7719149]
- 3.
- Majzoub JA, Muglia LJ. Knockout mice. N Engl J Med. 1996;334:904–907. [PubMed: 8596575]
- 4.
- Rubin EM, Barsh GS. Biological insights through genomics: mouse to man. J Clin Invest. 1996;97:275–280. [PMC free article: PMC507015] [PubMed: 8567945]
- 5.
- Thomas KR. The knockout mouse: six yeras old and growing stronger. Am J Respir Cell Mol Biol. 1995;12:461–463. [PubMed: 7742010]
- 6.
- Pulsinelli WA, Brierley JB, Plum F. Temporal profile of neuronal damage in a model of transient forebrain ischemia. Ann Neurol. 1982;11:491–498. [PubMed: 7103425]
- 7.
- Fujii M, Hara H, Meng W. et al. Strain-related differences in susceptibility to transient forebrain ischemia in SV-129 and CC57/balck/6 mice. Stroke. 1997;28:1805–1811. [PubMed: 9303029]
- 8.
- Tamura A, Graham DI, McCulloch J. et al. Focal cerebral ischemia in the rat: 1. Desprrcition of technique and early neuropathological consequences following middle cerebral artery occlusion. J Cereb Blood Flow Metab. 1:53–60. [PubMed: 7328138]
- 9.
- Tamura A, Graham DI, McCulloch J. et al. Focal cerebral ischemia in the rat. 2. Regional cerebral bloow flow determined by [14C]iodoantopyrine autoradiography following middle cerebral artery occlusion. J Cereb Blood Flow Metab. 1:61–69. [PubMed: 7328139]
- 10.
- Belayev L, Busto R, Zhao W. et al. HU-211, a novel noncompetitive N-methyl-D aspartate antagonist, improves neurological deficit and reduces infarct volume after reversible focal cerebral ischemia in the rat. Stroke. 1995;26:2313–2320. [PubMed: 7491657]
- 11.
- Brint S, Jacewicz M, Kiessling M. et al. Focal brain ischemia in the rat: methods for reproducible neocortical infarction using tandem occlusion of the distal middle cerebral artery and ipsilateral common carotid arteries. J Cereb Blood Flow Metab. 1988;8:474–485. [PubMed: 3392112]
- 12.
- Hara H, Huang PL, Panahian N. et al. Reduced brain edema and infarction volume in mice lacking the neuronal isoform of nitric oxide synthase after transient MCA occlusion. J Cereb Blood Flow Metab. 1996;16:605–611. [PubMed: 8964799]
- 13.
- Hallenbeck JM, Dutka AJ. Background review and current concept of reperfusion injury. Arch Neurol. 1990;47:1245–1254. [PubMed: 2241624]
- 14.
- Ames A I I I, Wright RL, Kowada M. et al. Cerebral ischemia II. the no-reflow phenomenon. Am J Pathol. 1968;52:437–453. [PMC free article: PMC2013326] [PubMed: 5635861]
- 15.
- Murakami K, Kondo T, Chan PH. Reperfusion following focal cerebral ischemia alters distribution of neuronal cells with DNA fragmentation in mice. Brain Res. 1997;751:160–164. [PubMed: 9098582]
- 16.
- Leist M, Vollbracht C, Kühnle S. et al. Caspase-mediated apoptosis in neuronal excitotoxicity triggered by nitric oxide. Mol Med. 1997;3:750–764. [PMC free article: PMC2230245] [PubMed: 9407551]
- 17.
- Bonfoco E, Krainc D, Ankarcrona M. et al. Apoptosis and necrosis: Two distinct events induced, respectively, by mild and intense insults with N-methyl-D-aspartate or nitric oxide/superoxide in cortical cell cultures. Proc Natl Acad Sci USA. 1995;92:7162–7166. [PMC free article: PMC41299] [PubMed: 7638161]
- 18.
- Du C, Hu R, Csernansky CA, Hsu C. et al. Very delayed infarction after mild focal cerebral ischemia: a role for apoptosis? J Cereb Blood Flow Metab. 1996;16:195–201. [PubMed: 8594050]
- 19.
- Endres M, Namura S, Shimizu-Sasamata M. et al. Attenuation of delayed neuronal death after mild focal cerebral ischemia in mice by inhibitors of the caspase family. J Cereb Blood Flow Metab. 1998;18:238–247. [PubMed: 9498840]
- 20.
- Fink K, Zhu J, Namura S. et al. Prolonged therapeutic window for ischemic brain damage caused by delayed caspase activation. J Cereb Blood Flow Metab. 1998;18:1071–1076. [PubMed: 9778183]
- 21.
- Dawson VL, Kizushi VM, Huang PL. et al. Resistance to neurotoxicity in cortical cultures from neuronal nitric oxide synthase-deficient mice. J Neurosci. 1996;16:2479–2487. [PMC free article: PMC6578778] [PubMed: 8786424]
- 22.
- Rothman S. Synapotic release of excitatory amino acid neurotransmitter mediates anoxic neuronal death. J Neurosci. 1984;4:1884–1891. [PMC free article: PMC6564878] [PubMed: 6737044]
- 23.
- Harms H, Wiegand F, Megow D. et al. Acute treatment of hypertension increases infarct size in spontaneously hypertensie rats. Neuroreport. 2000;11:355–359. [PubMed: 10674486]
- 24.
- Dirnagl U, Iadecola C, Moskowitz MA. Pathobiology of ischaemic stroke. An integrated view. Trends Neurosci. 1999;22:391–397. [PubMed: 10441299]
- 25.
- Martin RL, Lloyd HG, Cowan AI. The early events of oxygen and glucose deprivation: Setting the ecence for neuronal death? Trends Neurosci. 1994;17:251–2578. [PubMed: 7521086]
- 26.
- Choi DW. Ischemia-induced neuronal apoptosis. Curr Opin Neurobiol. 1996;6:667–672. [PubMed: 8937832]
- 27.
- Chen H, Chopp MN, Vande Linde M Q V. et al. The effects of post. ischemic hypothermia on the neuronal injury and brain metabolism after forebrain ischemia in the rat. J Neurol Sci. 1992;107:191–198. [PubMed: 1564517]
- 28.
- Furukawa K, Fu W, Li Y. et al. The actin-severing protein gelsolin modulates calcium channel and NMDA receptor activities and vulnerability to excitotoxicity in hippocampal neurons. J Neurosci. 1997;17:8178–8186. [PMC free article: PMC6573728] [PubMed: 9334393]
- 29.
- Endres M, Fink KB, Zhu J. et al. Neuroprotective effects of gelsolin during murine stroke. J Clin Invest. 1999;103:347–354. [PMC free article: PMC407902] [PubMed: 9927495]
- 30.
- Beckman JS, Koppenol WH. Nitric oxide, superoxide, and peroxynitrite: the good, the bad, and ugly. Am J Physiol. 1997;271:C1424–1437. [PubMed: 8944624]
- 31.
- Iadecola C. Bright and dark sides of nitric oxide in ischemic brain injury. Trends Neurosci. 1997;20:132–139. [PubMed: 9061868]
- 32.
- Dalkara T, Moskowitz MA. The complex role ot nitric oxide in the pathophysiology of focal cerebral ischemia. Brain Pathol. 1994;4:49–57. [PubMed: 7517769]
- 33.
- Zhang J, Dawson VL, Dawson TM. Nitric oxide activation of poly(ADP-ribose)synthetease in neurotoxicity. Science. 1994;263:687–689. [PubMed: 8080500]
- 34.
- Szabo C, Zingarelli B, O'Connor M. et al. DNA strand breakage, activation of poly(ADP-ribose)synthetase, and cellular energy depletion are involved in the cytotoxicity of macrophages and smooth muscle cells exposed to peroxynitrite. Proc Natl Acad Sci. 1996;93:1753–1758. [PMC free article: PMC39853] [PubMed: 8700830]
- 35.
- Endres M, Wang Z -Q, Namura S. et al. Ischemic brain injury is mediated by the activation of poly(ADP-ribose)polymerase. J Cereb Blood Flow Metab. 1997;17:1143–1151. [PubMed: 9390645]
- 36.
- Eliasson M J L, Sampei K, Mandir AS. et al. Poly(ADP-ribose)polymerase gene disruption renders mice resistant to cerebral ischemia. Nature Med. 1997;3:1089–1095. [PubMed: 9334719]
- 37.
- Endres M, Scott GS, Namura S. et al. Role of perosynitrite and neuronal nitric oxide synthase in the activation of poly(ADP-ribose)synthetase, against peroxynitrite-induced glial dmaage and stroke development. Neurosci Lett. 1998;248:41–44. [PubMed: 9665659]
- 38.
- Huang PL, Huang Z, Mashimo H. et al. Hypertension in mice lacking the gene for endothelial nitric oxide snythase. Nature. 1995;377:239–242. [PubMed: 7545787]
- 39.
- Huang Z, Huang PL, Ma J. et al. Enlarged infarcts in endothelial nitric oxide synthase knockout mice are attenuated by nitro-L-arginine. J Cereb. Blood Flow Metab. 1996;16:981–987. [PubMed: 8784243]
- 40.
- Endres M, Laufs U, Huang Z. Stroke protection by 3-hydroxy-3 methylglutaryl (HMG)-CoA reductase inhibitors mediated by endothelial nitric oxide snythase. Proc Natl Acad Sci USA. 1998;95:8880–8885. [PMC free article: PMC21171] [PubMed: 9671773]
- 41.
- Laufs U, Gertz K, Huang PL. et al. Atorvastatin upregulates type III nitric oxide synthase in thrombocytes, decreases platelet activation, and protects from stroke in normocholesterolemix mice. Stroke. 31:2437–2449.
- 42.
- Dugan LL, Choi DW. Excitotoxicity, free radicals, and cell membrane changes. Ann Neurol. 1994;35 Suppl:S17–21. [PubMed: 8185290]
- 43.
- Kristian T, Siesjo BK. Calcium in ischemic cell death. Stroke. 1998;29:705–718. [PubMed: 9506616]
- 44.
- Fujimura M, Morita-Fujimura Y, Murakami K. et al. Cytosolic redristribution of cytochrome c after transient focal cerebral ischemia in rats. J Cereb Blood Flow Metab. 1998;18:1239–1247. [PubMed: 9809513]
- 45.
- Siesjö BK, Kristian T. Katsura In: Ginsberg MD, Bogousslavsky J, Eds. Cerebrovascular Disease Blackwell Science, 19981–13.
- 46.
- Hossmann KA. Periinfarct depolarizations. Cerebrovasc Brain Metab Rev. 1996;8:195–208. [PubMed: 8870974]
- 47.
- Obrenovitch TP. The ischaemic penumbra: Twenty years on. Cerebrovasc Brain Metab Rev. 1995;7:297–323. [PubMed: 8703672]
- 48.
- Astrup J, Siesjo BK, Symon L. Thresholds in cerebral ischemiaThe ischemic penumbra. Stroke. 1981;12:723–725. [PubMed: 6272455]
- 49.
- Furlan M, Marchal G, Viader F. et al. Spontaneous neurological recovery after stroke and the fate of the ischemic penumbra. Ann Neurol. 1996;40:216–226. [PubMed: 8773603]
- 50.
- Read SJ, Hirano T, Abbott DF. et al. Identifying hypoxic tiossue after acute ischemic stroke using PET and 18F-fluoromisonidazole. Neurology. 1998;51:1617–1621. [PubMed: 9855512]
- 51.
- Kaufmann AM, Firlik AD, Fukui MB. et al. Ischemic core and penumbra in human stroke. Stroke. 1999;30:93–99. [PubMed: 9880395]
- 52.
- Deutsch SI, Huntzinger JA, Rosse RB. et al. The role of excitatory amino acids and intraneuronal calcium in the acute intoxicational effects of ethanol. Clin Neuropharmacol. 1989;12:1–13. [PubMed: 2557974]
- 53.
- Olney JW, Labruyere J, Price MT. Pathological changes induced in cerebrocortical neurons by phencyclidine and related drugs. Science. 1989;244:1360–1362. [PubMed: 2660263]
- 54.
- Turski L, Huth A, Sheardown M. et al. ZK20075: a phosphonate quinoxalinedione AMPA antagonist for neuroprotection in stroke and trauma. Proc Natl Acad Sci USA. 1998;95:10960–10965. [PMC free article: PMC28003] [PubMed: 9724812]
- 55.
- Bond A, O'Neill MJ, Hicks et al. Neuroprotective effects of a systemically active group II metabotropic glutatmate receptor agonist LY354740 in a gerbil model of global ischemia. Neuroreport. 1998;9:1191–1193. [PubMed: 9601692]
- 56.
- Nedergaard M, Hansen AJ. Characterization of cortical depolarizations evoked in focal cerebral ischemia. J Cereb Blood Flow Metab. 1993;13:568–574. [PubMed: 8314912]
- 57.
- Haring HP, Berg EL, Tsurushita N. et al. E-selectin apperas in nonischemic tissue during experimental focal ischemia. Stroke. 1996;27:1386–1391. [PubMed: 8711807]
- 58.
- Mies G, Iijima T, Hossmann KA. Correlation between peri-infarct DC shift and ischaemic neuronal damage in rat. NeuroReport. 1993;4:709–711. [PubMed: 8347812]
- 59.
- Iijima T, Mies G, Hossmann KA. Repeated negative DC deflections in rat cortex following middle cerebral artery occlusion are abolished by MK-801: Effect on volume of ischemic injury. J Cereb Blood Flow Metab. 1992;12:727–733. [PubMed: 1506440]
- 60.
- Back T, Hirsch JG, Szabo K. et al. Failure to demonstrate peri-infarct depolarizations by repetitive MR diffusion imaging in acute human stroke. Stroke. 2000;31:2901–2906. [PubMed: 11108746]
- 61.
- O'Neill LA. Kaltschmidt . NF-kappa B: A crucial transcription factor for glial and neuronal cell function. Trends Neurosci. 1997;20:252–258. [PubMed: 9185306]
- 62.
- Ruscher K, Isaev N, Trendelenburg G. et al. Induction of hypoxia inducible factor 1 by oxygen glucose deprivation is attenuated by hypoxic preconditioning in rat cultured neurons. Neurosci Lett. 1998;254:117–120. [PubMed: 9779934]
- 63.
- Iadecola C, Zhan F, Casey R. et al. Delayed reduction of ischemic brain injury and neurological deficits in mice lacking the inducible nitric oxide synthase gene. J Neurosci. 1997;17:9157–9164. [PMC free article: PMC6573582] [PubMed: 9364062]
- 64.
- Lindsberg PJ, Carpen O, Paetau A. et al. Endothelial ICAM-1 expression associated with inflammatory cell response in human ischemic stroke. Circulation. 1996;94:939–945. [PubMed: 8790029]
- 65.
- Pirttilä TR, Kauppinen RA. Recovery of intracellular pH in cortical brain slices following anoxia studied by nuclear magnetic resonance spectroscopy: Role of lactate removal, extracellular sodium and sodium/hydrogen exchange. Neuroscience. 1992;47:155–164. [PubMed: 1315933]
- 66.
- Gong C, Qin Z, Betz AL. et al. Cellular localization of tumor necrosis factor alpha following focal cerebral ischemia in mice. Brain Res. 1998;801:1–8. [PubMed: 9729236]
- 67.
- Feuerstein GZ, Wang X, Barone FC. Pathophysiology, diagnosis and management In: Ginsberg MD, Bogousslavsky J, Eds. Cerebrovascular Disease Malden: Blackwell Science, 1998507–531.
- 68.
- Chopp M, Li Y, Jiang N. et al. Antibodies against adhesion molecules reduce apoptosis after transient middle cerebral aretry occlusion in rat brain. J Cereb Blood Flow Metab. 1996;16:578–584. [PubMed: 8964796]
- 69.
- Dereski MO, Chopp M, Knight RA. et al. the heterogeneous temporal evolution of focal ischemic neuronal damage in the rat. Acta Neuropathol (Berl). 1993;85:327–333. [PubMed: 8460534]
- 70.
- Baird AE, Benfield A, Schlaug G. et al. Enlargment of human cerebral ischemic lesion volumes measured by diffusion-weighted magnetic resonance imaging. Ann Neurol. 1997;41:581–589. [PubMed: 9153519]
- 71.
- Iadecola C, Niwa K, Nogawa S. et al. Reduced susceptibility to ischemic brain injury and N-methyl-D-aspartate-mediated neurotoxicity in cyclooxygenase-2-deficient mice. Proc Natl Acad Sci USA. 2001;98:1294–1299. [PMC free article: PMC14748] [PubMed: 11158633]
- 72.
- Barone FC, Arvin B, White RF. et al. Tumor necrosis factor-alpha. a mediator of focal ischemic brain injury. Stroke. 1997;28:1233–1244. [PubMed: 9183357]
- 73.
- Bruce AJ, Boling W, Kindy MS. et al. Altered neuronal and microglial responses to excitotoxic and ischemic brain injury in mice lacking TNF receptors. Nat Med. 1996;2:788–794. [PubMed: 8673925]
- 74.
- Kerr JF, Wyllie AH, Currie AR. Apoptosis: a basic biological phenomenen with wide-ranging implication in tissue kinetics. Br J Cancer. 1972;26:239. [PMC free article: PMC2008650] [PubMed: 4561027]
- 75.
- Linnik MD, Zobrist RH, Hatfield MD. Evidence supporting a role for programmed cell death in focal cerebral ischemia in rats. Stroke. 1993;24:2002–2009. [PubMed: 8248983]
- 76.
- Tominaga T, Kure S, Narisawa K. et al. Endonuclease activation following focal ischemic injury in the rat. Brain Res. 1993;608:21–26. [PubMed: 8388311]
- 77.
- MacManus JP, Buchan AM, Hill IE. et al. Global ischemia can cause DNA fragmentation indicative of apoptosis in rat brain. Neurosci Lett. 1993;164:89–92. [PubMed: 8152622]
- 78.
- Majno G, Joris I. Apoptosis, oncosis, and necrosis. An overview of cell death. Am J Pathol. 1995;146:3–15. [PMC free article: PMC1870771] [PubMed: 7856735]
- 79.
- MacManus JP, Hill IE, Huang ZG. et al. DNA damage consistent with apoptosis in transient focal ischaemic cortex. NeuroReport. 1994;5:493–496. [PubMed: 8003682]
- 80.
- MacManus JP, Hill IE, Preston E. et al. Differences in DNA fragmentation following transient cerebral or decapitation ischemia in rats. J Cereb Blood Flow Metab. 1995;15:728–737. [PubMed: 7673368]
- 81.
- Charriaut-Marlangue C, Margaill I, Plotkine M. et al. Early endonuclease activation following reversible focal ischemia in the rat brain. J Cereb Blood Flow Metab. 1995;15:385–388. [PubMed: 7713995]
- 82.
- Charriaut-Marlangue C, Margaill I, Represa A. et al. Apoptosis and necrosis after reverisble focal ischemia: an in situ DNA fragmentation analysis. J Cereb Blood Flow Metab. 1996;16:186–194. [PubMed: 8594049]
- 83.
- Li Y, Chopp M, Jiang N. et al. In situ detection of DNA fragmentation after focal cerebral ischemia in mice. Mol Brain Res. 1995;28:164–168. [PubMed: 7707871]
- 84.
- Li Y, Chopp M, Jiang N. et al. Induction of DNA fragmentation after 10 to 120 min of focal cerebral ischemia in rats. Stroke. 1995;26:1252–1258. [PubMed: 7541573]
- 85.
- van Lookeren Campagne M, Gill R. Ultrastructural morphological changes are not characteristic of apoptototic cell death following focal cerebral ischaemia in the rat. Neurosci Lett. 1996;213:111–114. [PubMed: 8858621]
- 86.
- Ameisen JC. The origin of programmed cell death. Science. 1996;272:1278–1279. [PubMed: 8650538]
- 87.
- 88.
- Hockenberry D. Defining apoptosis. Am J Pathol. 1995;146:16–19. [PMC free article: PMC1870758] [PubMed: 7856725]
- 89.
- Raff MC, Barres BA, Burne JF. et al. Programmed cell death and the control of cell survival: Lessons from the nervous system. Science. 1993;262:695–700. [PubMed: 8235590]
- 90.
- Chinaiyan Am, O'Rourke K, Lane Br. et al. Interaction of CED-4 with CED-3 and CED.-9: A molecular framework for cell death. Science. 1997;275:1122–1126. [PubMed: 9027312]
- 91.
- Ellis HM, Horvitz HR. Genetic control of programmmed cell death in the nematode C. elegans. Cell. 1986;44:817–829. [PubMed: 3955651]
- 92.
- Ellis HM, Yuan J, Horvitz HR. Mechanisms and functions of cell death. Annu Rev Cell Biol. 1991;7:663–698. [PubMed: 1809356]
- 93.
- Wu DG, Wallen WD, Nunez G. Interaction and regulation of subcellular localization of CED-4 by CED-9. Science. 1997;275:1126–1129. [PubMed: 9027313]
- 94.
- Yuan J, Shaham S, Ledoux S. et al. The C. elegans cell death gene ced-3 encodes a protein similar to mammalian interleukin-1 beta-converting enzyme. Cell. 1993;75:641–652. [PubMed: 8242740]
- 95.
- Alnemri ES, Livingston DJ, Nicholson DW. et al. Human ICE/CED-3 protease nomenclature. Cell. 1996;87:171. [PubMed: 8861900]
- 96.
- Redd CJ. Cytochrome c: Can't live itCan't live without it. Cell. 1997;91:559–562. [PubMed: 9393848]
- 97.
- Namura S, Zhu J, Fink K. et al. Activation and cleavage of caspase-3 in apoptosis induced by experimental cerebral ischemia. J Neurosci. 1998;18:3659–3668. [PMC free article: PMC6793169] [PubMed: 9570797]
- 98.
- Friedlander RM, Gagliardini V, Hara H. et al. Expression of a dominant negative mutant of interleukin-1 beta converting enzyme in trasngenic mice prevents neuronal death induced by trophic factor withdrawal and ischemic brain injury. J Exp Med. 1997;185:933–940. [PMC free article: PMC2196165] [PubMed: 9120399]
- 99.
- Schielke GP, Yang GY, Shivers BD. et al. Reduced ischemic brain injury in interleukin-1 beta converting enzyme-deficient mice. J Cereb Blood Flow Metab. 1998;18:180–185. [PubMed: 9469161]
- 100.
- Hara H, Friedlander RM, Gagliardini V. et al. Inhibition of interleukin 1beta converting enzyme family proteases reduces ischemic and excitotoxic neuronal damage. Proc Natl Acad Sci USA. 1997;94:2007–2012. [PMC free article: PMC20033] [PubMed: 9050895]
- 101.
- Shaw E. Cysteinyl proteinases and theirr selective inactivation. Adv Enzymol Relat Areas Mol Biol. 1990;63:271–347. [PubMed: 2407065]
- 102.
- Sarin A, Wu ML, Henkart P. Different interleukin-1 beta converting enzyme family protease requirements for the apoptotic death of T lymphocytes triggered by diverse stimuli. J Exp Med. 1996;184:2445–2450. [PMC free article: PMC2196389] [PubMed: 8976202]
- 103.
- Nicholson WD, Ali A, Thornberry NA. et al. Identification and inhibition of the ICE/CED-3 protease necessary for mammalian apoptosis. Nature. 1995;376:37–43. [PubMed: 7596430]
- 104.
- Nicholson WD, Thornberry NA. Caspases: killer proteases. Trends Biochem Sci. 1997;22:299–306. [PubMed: 9270303]
- 105.
- Chen J, Nagayama T, Jin K. et al. Induction of caspase-3-like protease may mediate delayed neuronal death in the hippocampus after transient cerebral ischemia. J Neurosci. 1998;18:4914–4928. [PMC free article: PMC6792571] [PubMed: 9634557]
- 106.
- Cheng Y, Deskmukh M, D-Costa A. et al. Caspase inhibitor affords neuroprotection with delayed administration in a rat model of neonatal hypoxic-ischemic brain injury. J Clin Invest. 1998;101:1992–1999. [PMC free article: PMC508786] [PubMed: 9576764]
- 107.
- Ma J, Endres M, Moskowitz MA. Synergistic effects of caspase inhibitors and MK-801 in brain injury after transient focal cerebral ischaemia in mice. Br J Pharmacol. 1998;124:756–762. [PMC free article: PMC1565432] [PubMed: 9690868]
- 108.
- Minamisawa H, Mellergard P, Smith ML. et al. Preservation of brain temperature during ischemia in rats. J Cereb Blood Flow Metab. 1990;10:365–374. [PubMed: 2339456]
- 109.
- Busto R, Globus M Y T, Dietrich D. et al. Small differences in intraischemic brain temperature critically determine the extent of ischemic neuronal injury. J Cereb Blood Flow Metab. 1987;7:729–738. [PubMed: 3693428]
- 110.
- Crumrine RC, LaManna JC. Regional cerebral metabolites, blood flow, plasma volume, and mean transit time in total cerebral ischemia in the rat. J Cereb Blood Flow Metab. 1991;11:272–282. [PubMed: 1997498]
- Epidemiological Data
- Introduction
- Global vs. Focal Ischemia
- Animal Models of Cerebral Ischemia
- In Vitro Models of Cerebral Ischemia
- Importance of Physiologic Parameters for Stroke Outcome
- Pathophysiological Cascades Following Cerebral Ischemia
- Glutamate Receptors and Excitotoxicity
- Tissue Acidosis
- Protein Synthesis and Early Gene Expression
- Molecular Mechanisms
- Experimental Evidence for Caspase-Mediated Cell Death Following Cerebral Ischemia
- Caspase Inhibition Protects From Cerebral Ischemia
- Conclusion
- References
- Neuroprotective Strategies in Animal and in Vitro Models of Neuronal Damage: Isc...Neuroprotective Strategies in Animal and in Vitro Models of Neuronal Damage: Ischemia and Stroke - Madame Curie Bioscience Database
- Regulation of Voltage-Sensitive Ca2+ Channels in Bipolar Cells by Divalent Catio...Regulation of Voltage-Sensitive Ca2+ Channels in Bipolar Cells by Divalent Cations and Polyamines - Madame Curie Bioscience Database
- DNA Primer Extension by Telomerase - Madame Curie Bioscience DatabaseDNA Primer Extension by Telomerase - Madame Curie Bioscience Database
- Caspase-Independent Cell Death Mechanisms - Madame Curie Bioscience DatabaseCaspase-Independent Cell Death Mechanisms - Madame Curie Bioscience Database
- Neurotrophins - Madame Curie Bioscience DatabaseNeurotrophins - Madame Curie Bioscience Database
Your browsing activity is empty.
Activity recording is turned off.
See more...