NCBI Bookshelf. A service of the National Library of Medicine, National Institutes of Health.
Madame Curie Bioscience Database [Internet]. Austin (TX): Landes Bioscience; 2000-2013.
The Sec translocase or translocon is the essential and ubiquitous system for protein translocation across or into the membrane. The core channel, the SecYE complex, is conserved across biological kingdoms and most of the polypeptide chains which are routed to extracellular or membrane locations in bacteria use this pathway. Biochemical and genetic approaches have yielded a substantial body of information about functional aspects of Sec-mediated translocation and this information has recently been enriched with structural data at atomic resolution. This chapter reviews previously acquired facts and concepts concerning the Sec translocase of bacteria in light of recent structural results and considers implications of these findings.
Introduction
In E. coli, components of the Sec pathway were identified during the mid 1980s using elegant genetic screens. Conditional-lethal mutations associated with a generalized protein-secretion defect or mutations restoring translocation of proteins with secretion-defective leader peptides allowed the identification of most of the Sec components.1-3 The translocation pathway was then successfully reconstituted in vitro in the early 1990s to allow biochemical dissection of the subreactions of the translocation event.4 It was shown that targeting of the protein substrate to the translocase is mediated by the dedicated chaperone SecB or by the signal recognition particle (SRP).5-7 The biochemical analysis showed further that the translocon is comprized of a membrane-embedded SecYE channel complex8 and a peripheral SecA ATPase which functions as a motor to drive translocation (fig. 1).9 Genomic analysis revealed that SecYE is highly conserved in bacteria, archaea and eukaryotes.10,11 Isolation of large amounts of SecYE complex or its eukaryotic homolog, the Sec61αγ complex, allowed for further biochemical, biophysical and structural analysis. Both complexes copurify with a small subunit, SecG and Sec61β respectively, although these subunits do not share obvious homology.11 Additional components, such as the heterotrimeric complex SecDFyajC12 and the proton motive force (PMF),13 were found to contribute to the Sec pathway but in vitro reconstitution experiments demonstrated that SecA, SecE and SecY are necessary and sufficient for the basal activity of the translocase.14,15 The following paragraphs describe in finer detail more recent findings and address current questions on the bacterial translocation system.
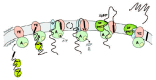
Figure 1
Model for preprotein translocation across the cytoplasmic membrane. See text for details.
The SecYEG Translocon at the Atomic Level
Biochemical, biophysical and electrophysiology studies established early on that the Sec complex serves as the channel through which preproteins traverse the membrane. The recent solution of the two-dimensional16,17 and three-dimensional18 structures of SecYEG (∼75 kDa) and SecYEβ from the bacterium E. coli and the archaea M. jannaschii, respectively, provide structural support and new insights for the translocation mechanism. As a full chapter on the Sec channel structure appears elsewhere in this book, only a brief description is given here (fig. 2). The SecY subunit consists of two sub-domains, the transmembrane segments TM1—TM5 and TM6—TM10, arranged like a clamp and related to each other by a two-fold pseudo-symmetry axis. The essential SecE-subunit docks its TM helix across the interface of the two SecY-domains, clamping them together. The proposed translocation channel is located in the center of the Y-subunit which is filled by a short distorted helix (TM2a, termed the plug) extending halfway to the center of the membrane. Movement of the plug would yield a continuous aqueous channel through which preproteins would be translocated. Halfway across the membrane plane, the channel is also constricted by a ring of six hydrophobic amino acid residues. This ring is proposed to seal the channel but would also widen just enough, probably by shifts in the helices forming the channel, to allow the passage of a polypeptide chain. The small Secβ subunit (SecG-like subunit) is peripherally attached and makes limited contact with SecY, consistent with its nonessential role in translocation. A groove situated between the TM segments at the edges of the two SecY halves (interface between TM2 and TM7) is accessible to the lipid bilayer. As the other sides of SecY are contacted by the SecE and Secβ subunits, this groove may form a lateral gate for release of TM segment of membrane protein.
Binding and Orientation of the Leader Peptide into the Translocon
Leader peptides consist of a short positively charged N-teminal region followed by a central hydrophobic core and a leader peptidase cleavage site. The physicochemical properties of leader peptides are essential for correct interaction with the translocon, such that the N terminus of the leader peptide stays in the cytosol while the hydrophobic core crosses the membrane. 19-21 Insertion of the leader peptide into the channel at an early stage of translocation has been thoroughly analyzed by photo-crosslinking technologies. With photoreactive probes positioned at single sites along the leader peptide, it was shown that the opposite sides of the hydrophobic core contact TM segments 2 and 7 of the Y-subunit.22-24 Each residue of the leader peptide could also be cross-linked to phospholipids, suggesting that the binding site is located at the interface of the protein channel and the lipid phase.23,25 These earlier experiments are now supported by the atomic structure of SecY which reveals that TM2 and TM7 are located at the interface of the two SecY halves, adjacent to the pore channel and accessible both from the lipid and cytoplasmic side of the membrane.18 Moreover, the sequences of TM domains 2 and 7 are well-conserved, suggesting a similar mechanism of leader peptide recognition across evolution.11 Binding and orientation of the leader peptide into the translocon also involves specific charged residues in the cytoplasmic and periplasmic loops of SecY. Site-directed charge-reversal mutations indicated that these conserved amino-acyl residues functionally interact with charged residues in the N-terminus of the leader peptide in order to set its correct topology in the channel.26,27 The residues immediately after the signal sequence were found in contact with the SecY-subunit but not with lipids, supporting a model in which the polypeptide chain inserts in a loop-like configuration into the channel.23
Opening of the Translocation Channel
Pioneering experiments showed that the addition of synthetic leader peptides to the cytoplasmic side of reconstituted E. coli membrane bilayers open aqueous pores detectable by conductivity measurements.28 The model derived from the atomic structure predicts that two movements may occur in order for the channel to open and to accommodate the leader and attached polypeptide.18 First, the plug domain may move away from its blocking position on the protein channel and second, the N- and C-terminal halves of SecY may move apart to create a lateral opening of the translocation pore necessary to embrace the polypeptide chain. Alternatively, a diaphragm-like movement of the TM segments would widen the pore sufficiently to allow insertion of the polypeptide chain. Although these putative movements await experimental support, some earlier experiments are in favor of these models. It was shown that unique cysteines introduced in the domain of SecY forming the plug (TM2a) and at the C-terminal end of SecE can form a disulfide bridge.29 Since these two cysteines are 20Å apart in the closed channel structure, the observed cross-link is now explained by the movement of the plug away from the center of the channel. Moreover, the disulfide bridge formation had a dominant lethal affect,29 as expected if the channel was locked into a permanently open state by the covalent modification. Other possible experimental support is provided by Prl mutants, a collection of mutations in SecY or SecE which up-regulate the activity of the translocase. Since these mutations allow secretory proteins with defective or even deleted leader peptides to be transported,30,31 they may mimic the effect of signal sequence binding. A previous study indicated that the Prl mutations increase the conformational flexibility of the translocon,32 and the atomic structure shows that most of the mutations are located in the center of the channel, particularly on the internal side of TM7 and in the plug.18 Thus, it is postulated the Prl mutations could increase the dynamics of the plug movement or facilitate widening of the pore during initiation of translocation, and therefore reduce the requirement for a functional leader peptide.
Translocation Pause
Short hydrophobic stretches in the mature domain of preproteins induce a transient pause in the translocation movement which leads to the formation of translocation intermediates across the channel.33 Deletion or relocation of these hydrophobic segments significantly alters the pattern of intermediates, while increasing the length and hydrophobicity of the stretch can lead to complete translocation arrest.34,35 These observations suggest that the mechanism involved during translocation pause and translocation arrest are probably similar. The atomic structure shows that the channel is shaped as an hourglass with a constriction of hydrophobic residues in its center. It is proposed that the hydrophobic ring may form a seal around the translocating polypeptide chain while the hourglass shape may serve to limit the contact of the chain with the channel walls.18 While organization may minimize the energy required for polypeptide movement through the membrane, it may also serve in the recognition of hydrophobic stretches. If the length and hydrophobicity of the stretch is sufficient to span the membrane, the protein is eventually released into the lipid phase of the membrane.35,36 The TM segment of a nascent membrane protein has been shown to move from the aqueous interior of the channel to the hydrophobic environment of the lipid bilayer.25,37 The atomic structure shows that the TM2/TM7 interface is the only possible escape for lateral release of TM segments of membrane proteins in transit through the translocon.
The Quaternary Structure of the SecYEG Translocon
The understanding of the translocation channel is further complicated by the fact that SecYEG exists as dimeric and tetrameric assemblies. Low resolution electron microscopy (EM) images of purified mammalian, yeast and bacterial translocon all revealed the oligomeric state of the Sec complex.38-41 The stoichiometry of these assemblies was then established using various biochemical and biophysical investigations such as crosslinking,42 sedimentation analysis16 and blue-native gel electrophoresis.43 In the SecYEG dimer, SecE is located at the interface of the protomers, such that the TM2/TM7 interfaces are pointed in opposite directions and toward the lipid bilayer. This is the organization seen in the 2D crystal structure,17 which is also supported by a cysteine crosslinking study showing that the two SecE subunits are close to one another.44 The organization of the Sec protomers within the tetrameric assemblies is unknown. It is argued that two dimers, each with an organization similar to that observed in the lattice of the two-dimentional crystals, may associate in a side-by-side manner to form a tetramer.45 Such organization leaves the lateral gates oriented toward the lipid bilayer but other configuration are, nonetheless, possible. Since evidence for the existence of SecYEG as a monomer in the membrane is lacking, it is unclear why the SecYEG complex exist as an oligomer while a single copy of SecYEG seems to form the translocation channel. The central depression seen in the oligomeric ring-like structure was initially postulated to form a translocation pore.38-41 In the 2D crystals, the dimeric translocon presents a funnel-like cavity formed by adjacent protomers and closed on its periplasmic face.17 It is now proposed that the porelike structure formed by the tetramer or the cavity observed within the dimer in fact reflect a depression at the interface of the protomers rather than a true translocation channel.
Dynamic Behavior of SecYEG Oligomers
It is also unclear whether the Sec complex undergoes transitions in its oligomeric status as part of the translocation event. Native electrophoresis experiments show that SecYEG dimers reversibly dissociate into monomers in a detergent-dependent manner43 and a protein concentration-dependent equilibrium between Sec tetramers and monomers exists, as detected by analytical centrifugation.16 However, such dynamic association of Sec protomers takes place in detergent solution and may be different once the SecYEG complex is embedded in the phospholipid bilayer. The reconstitution of membranes containing active SecYEG involves the dilution of detergent and thus prompts the formation of dimers.43 The same is true for the growth of 2D crystals and only dimeric assemblies formed in the crystallized membrane.16 Moreover, subunit exchange studies46 and fluorescence resonance energy transfer experiments47 failed to observe any exchange of the protomeric components within the membranous oligomer. Altogether, these observations argue that the translocon may not experience rearrangements in its oligomeric status per se, although translocation-related dynamic changes may occur. Indeed, cysteine-scanning mutagenesis identified enhancement of the interhelical SecE contact at the initiation of translocation,42 suggesting that translocation results in a rearrangement of SecE molecules within the SecYEG oligomer. Electron microscopy analysis of proteoliposome-reconstituted, detergent-solubilized dimeric SecYEG revealed that binding of SecA with nucleotides lead to the recruitment of two SecYEG dimers to form the tetrameric SecYEG assembly.41 Similarly, reconstitution of the mammalian translocon ring structure required the presence of ribosomes for recruitment of individual Sec61 eukaryotic complexes.48
Atomic Structure of the SecA Translocation Motor
The SecA ATPase (∼100 kDa) interacts with the SecYEG channel to drive translocation. In addition to its high affinity for SecYEG,49 SecA also interacts with numerous ligands: leader and mature regions of preproteins, acidic phospholipids, SecB, nucleotides, Mg,2+ Zn2+ and its own mRNA.50 Accordingly, the crystal structure of SecA from B. subtilis reveals a complex multidomain protein (fig. 3).51 The motor ATPase domain is made up of two RecA-like folds (termed nucleotide binding folds, NBF) similar to those found in superfamily 1 and 2 helicases.52 The interface between NBF1 and NBF2 forms the nucleotide binding site. Three other domains are linked to the ATPase domain: the preprotein cross-link domain (PPXD), helical wing domain (HWD), and helical scaffold domain (HSD).51 The PPXD domain can be cross-linked to the leader and mature domain of preprotein.53,54 The HSD domain contains a long α-helix acting as a connection between the motor and translocation domains of SecA. The HWD domain is an insertion in the HSD domain and seems to be flexible and loosely linked with the rest of the molecule. The extreme C-terminal region (CTD) is also flexible and has been shown to bind lipid, Zn2+ and SecB.55,56 Recently, comparison of the atomic structures of monomeric and dimeric SecA revealed that SecA monomerization generates relative movement of the PPXD, HSD and HWD domains such that a potential preprotein-binding groove forms at the surface of SecA57 (see below also).
Binding of the SecA Motor to the SecYEG Channel
The regions of interaction between SecA and SecYEG remain to be characterized. Ligand affinity blotting experiments indicate that SecA binds to the first 107 amino acid residues of SecY58 and intergenic suppressor studies suggest that the C-terminal cytoplasmic loop (between TM8 and TM9) and the C-terminal tail of SecY are important for SecA interaction. 59-61 Mutations in the C-terminal tail do not abolish the binding of SecA, but prevents its activation.59 Similarly, random targeted mutagenesis identified residues in the cytoplasmic loop TM8/TM9 as indispensable for productive SecA-dependent translocation, but their effect on SecA binding is unknown.60 The sequences of SecA that interact with SecYEG are also poorly defined. Extragenic suppressors of SecY mutations map all over SecA molecules.62 Ligand affinity experiments identified the C-terminal third of SecA as the SecYEG-interacting domain.58 In contrast, characterization of the binding constant of truncated SecA derivatives indicate that the N-terminal domain comprizing the ATPase motor is responsible for the interaction with SecYEG63 but both domains may contribute to optimal binding of SecA to the SecYEG complex. Moreover, the stoichiometry of the SecA-SecYEG association is largely unknown (see below).
How Does SecA Use ATP to Catalyze Translocation?
The SecYEG-bound SecA ATPase activity is stimulated by a translocation-competent preprotein.9 This activity, termed SecA translocation ATPase, is responsible for the preprotein translocation reaction. In vitro reconstitution shows that the initiation step requires ATP binding but not its hydrolysis.64 This initial event leads the leader peptide and attached polypeptide to cross the channel in a loop-like configuration such that it can be processed by a signal peptidase at the periplasmic face of the membrane. Continued translocation then requires ATP hydrolysis which causes cycles of binding and the release of the preprotein from SecA.64,65 Under appropriate in vitro conditions, it has been shown that translocation is a stepwise proccess, corresponding to translocation steps of 20—30 amino acid residues of the polypeptidic chain.64,67 The mechanism by which the energy of ATP binding hydrolysis at SecA is converted into the movement of preproteins across the membrane has been related to the SecA transmembrane mobility at SecYEG, called the insertion—deinsertion cycle.68 This model is based on the observation that SecA becomes protected from added protease under the conditions of active preprotein translocation.68,69 Several regions of SecA are indeed accessible for chemical modification from the periplasmic side of the membrane.70-72 The membrane insertion-desinsertion of SecA is regulated by ATP and repeated cycles of these movements has been proposed to drive the stepwise movement of preprotein across the membrane.68 Whether SecA truly inserts into the translocon and across the membrane, and how the ATP-derived energy is coupled to the preprotein movement, remains controversial and not yet fully understood. What is clear is that SecA undergoes conformational changes that are coupled to its interaction with ligands and driven by the ATPase cycle. By analogy to the helicase working mechanism,52 the two RecA-like domains of SecA may move relative to one another during the ATPase cycle, creating domain movements which may be propagated via the long αhelix (HSD) to the other SecA domains to generate preprotein motion.73,74 Steady-state tryptophan fluorescence anisotropy spectroscopy suggests that nucleotide-free SecA is in a domain-dissociated conformation which may have high affinity for SecYEG.51 In contrast, nucleotide binding would result in the presentation of compact conformations with low affinity. Thus, both SecA conformational changes and variation in SecYEG-affinity would provide the driving force for translocation to occur.
The SecA Monomer-Dimer Equilibrium
An understanding of the SecA mechanism seems further complicated by the fact that SecA exists in solution as a dimer in equilibrium with a small fraction of monomers.75,76 The dimeric organization maximizes the buried solvent-accessible surface area and intermolecular protomeric contacts, but the interface between the SecA dimer is not extensive.51,57 The equilibrium can be shifted towards the monomeric state by relatively small changes in the SecA primary sequence or incubation conditions.75,77 An early study based on fluorescence resonance energy transfer experiments and involving heterodimers with one ATPase-inactive subunit has suggested that the SecA dimer is the active species in translocation,78 but this view has been recently challenged. Acidic lipids, which are essential for SecA activation were found to induce dissociation of the dimer.77 Furthermore, synthetic signal peptides can induce monomerization of SecA while a mutant that fails to dimerize retains some translocation activity. 77,79 Finally, the rotation of the PPXD domain, which generates a large groove probably involved in preprotein binding, occurs upon monomerization of SecA.57 Altogether, these recent observations suggest that the active form of SecA may be monomeric or that SecA monomerization may be critical at some stage of the translocation reaction. The oligomeric state of SecA during translocation and when bound at SecYEG remains, however, to be determined. Recent native electrophoresis and analytical centrifugation experiments suggest that detergent-solubilized and stabilized dimeric SecYEG can bind both monomeric and dimeric SecA with a stoichiometry modulated by nucleotides.80,81 The variability in the stoichiometry of the SecYEG-SecA complex might carry significant implications. It is now hypothesized that the mechanism by which SecA mediates protein translocation may resemble the mechanism by which helicases mediate unwinding of nucleic acid duplexes.82,83 Two distinct mechanisms, called the inch-worm model and the active rolling model, can be envisioned. According to the ‘inchworm’ mechanism, the SecA monomer is the functionally active species: cycles of ATP binding and hydrolysis would trigger localized conformational changes in the SecYEG-bound SecA monomer, leading to processive feeding of the polypeptide through the channel. According to the ‘rolling’ model, ATP-driven cycles of SecA monomerization-dimerization would mediate the processive passage of preprotein: a free SecA monomer may bind a new segment of preproteins before reassociating with the SecYEG-bound SecA monomer. In both models, a SecYEG-bound SecA monomer would be maintained in close association with the channel throughout the translocation process.
The Translocase Makes Use of the Proton Motive Force
Preprotein translocation is strongly stimulated by the PMF, both with native membranes and with purified and reconstituted SecYEG translocase.84,85 Several subreactions of the translocation process seem to be simultaneously affected by the PMF. Earlier studies have shown that PMF can drive forward movement of preprotein translocation intermediates when SecA is no longer associated with the polypeptidic chain.67,86 The Δψ and ΔpH components of the PMF may act on the preprotein itself via some sort of electrophoretic or folding effect on the polypeptide chain in transit.87 The binding of leader peptide to the cytoplasmic membrane and its subsequent insertion in the translocation channel may also be optimized by the PMF.89 Alternatively, or in addition, the PMF may directly modify the conformation of the translocation channel and its subsequent interaction with the translocation partners. Indeed, the Prl mutations which may alter the conformation of the channel render the in vivo and in vitro translocation less PMF-dependent, suggesting that Prl mutations may mimic the effect of the PMF.90 Furthermore, the PMF accelerates the conformational changes of SecA that occur during translocation91 and the stimulatory effect of the PMF is more obvious at low SecA concentration.92 The same Prl mutations, which decrease the PMF-dependency of translocation,90 also increase the affinity of SecA for SecYEG.93 It is thus possible that the PMF could change the conformation of the channel such that it modifies the dynamic of the SecYEG-SecA association.
Additional Subunits Make the Translocase Holo-Enzyme
In contrast to SecA, SecY and SecE, the SecG subunit is not essential for cell viability and translocation and is not conserved outside the bacteria kingdom.11 SecG is a 12-kDa protein with two TM segments connected via a short apolar cytosolic segment.94,95 This small subunit enhances the translocation rate and this enhancing effect is particularly seen in vitro. SecG is not needed for the high-affinity binding of SecA to SecYE but it readily stimulates SecA activity.13,96 The in vivo contribution of SecG is clearly observed only when SecA function is compromized by mutations or when SecA activity may become more critical for translocation such as at low temperatures, in the absence of SecDF, in absence of acidic phospholipids, or at low transmembrane PMF.13,97 It has been shown that SecG exists in two inverted topological states in the membrane and interconversion between these states is linked to the SecA membrane insertion reaction.98 SecG may enhance translocation and SecA activity by acting on the conformation of the translocation channel. However, the atomic structure shows that SecG is located at the periphery of the SecYE complex18 and EM pictures apparently indicate that SecG is not required for the formation of the SecYE ring-like structures.40
The core SecYE also associates with the SecDFyajC heterotrimeric membrane protein complex.13,99 SecD and SecF present sequence similarity, each spanning the membrane six times and possesing a large periplasmic loop between the first and second transmembrane segments.100 In B. subtilis, SecD and SecF are even fused into one large polypeptide.101 YajC, a small single transmembrane protein, exists in tight complex with SecDF13,99 and its gene is located in the same operon. Altogether, these observations suggest that the role of these three proteins is somehow linked but their true function remains largely unknown. In vivo, the absence of SecDF, but not YajC, severely affects cell viability and the efficiency of protein translocation.12 In vitro, the stimulatory effect of SecDFyajC is obvious only when membranes are depleted for SecG, suggesting that the stimulatory function of SecG covers that of SecDFyajC in the reconstituted system.15 Interestingly, the level of SecG in membranes is decreased upon SecDFyajC depletion and recovered to a normal level when SecDFyajC is expressed,102 suggesting that a coordinated balance between these stimulatory subunits exist. At low translocation rates, it has been shown SecDFyajC increases the formation and accumulation of preprotein translocation intermediates in transit across the channel.103 These translocation intermediates are then propelled forward after energization of the membrane by the PMF. This result suggests that SecDFyajC may serve to coordinate the action of ATP- and PMF-driven translocation.103 SecDFyajC has also been shown to modulate the behaviour of SecA toward stabilization of the membrane-inserted conformation.104 Since archaea contain SecD and SecF homologues while a SecA homologue is absent,101 this later effect may be indirect and rather caused by the stabilization of the translocation intermediate.103 Finally, it has been proposed that SecD plays a role in protein release following the translocation event.105
Concluding Remarks
Genetic and biochemical studies have provided the first elementary and essential insights in the mechanism of preprotein translocation. Structural studies have resulted in a significant advance of our understanding and allowed further interpretation of previously obtained experimental data. As discussed in several places in this chapter, many aspects of the translocation reaction are accompanied by dynamic conformational changes and transient associations. The combination of advanced biochemical tools together with high-resolution structural approaches will soon allow for an exact description of the relation of structure to function, leading to detailed knowledge about the mechanism of protein translocation across and into the cytoplasmic membrane. Finally, further challenges include the description of translocon function integrated in the larger context of the cell physiology, and its cross-talk with the other translocation systems present in the cell envelope.
Acknowledgments
We thank Dr. J. Brunstein for critical reading of the manuscript. FD greatfully acknowledges research support from the Canada Foundation for Innovation, the Canada Research Chair program and the University of British Columbia. APM is supported by a scholarship from INSERM-CIHR.
References
- 1.
- Danese PN, Silhavy TJ. Targeting and assembly of periplasmic and outer-membrane proteins in Escherichia coli. Annu Rev Genet. 1998;32:59–94. [PubMed: 9928475]
- 2.
- Bieker KL, Phillips GJ, Silhavy TJ. The sec and prl genes of Escherichia coli. J Bioenerg Biomembr. 1990;22:291–310. [PubMed: 2202721]
- 3.
- Schatz PJ, Beckwith J. Genetic analysis of protein export in Escherichia coli. Annu Rev Genet. 1990;24:215–248. [PubMed: 2088168]
- 4.
- Wickner W, Leonard MR. Escherichia coli preprotein translocase. J Biol Chem. 1996;271:29514–29516. [PubMed: 8939874]
- 5.
- Valent QA, Scotti PA, High S. et al. The Escherichia coli SRP and SecB targeting pathways converge at the translocon. EMBO J. 1998;17:2504–2512. [PMC free article: PMC1170592] [PubMed: 9564033]
- 6.
- Koch HG, Hengelage T, Neumann-Haefelin C. et al. In vitro studies with purified components reveal signal recognition particle (SRP) and SecA/SecB as constituents of two independent protein-targeting pathways of Escherichia coli. Mol Biol Cell. 1999;10:2163–2173. [PMC free article: PMC25430] [PubMed: 10397756]
- 7.
- Driessen AJ, Manting EH, van der Does C. The structural basis of protein targeting and translocation in bacteria. Nat Struct Biol. 2001;8:492–498. [PubMed: 11373615]
- 8.
- Brundage L, Fimmel CJ, Mizushima S. et al. SecY, SecE and band 1 form the membrane-embedded domain of Escherichia coli preprotein translocase. J Biol Chem. 1992;267:4166–4170. [PubMed: 1531482]
- 9.
- Lill R, Cunningham K, Brundage LA. et al. SecA protein hydrolyzes ATP and is an essential component of the protein translocation ATPase of Escherichia coli. EMBO J. 1989;8:961–966. [PMC free article: PMC400897] [PubMed: 2542029]
- 10.
- Hartmann E, Sommer T, Prehn S. et al. Evolutionary conservation of components of the protein translocation complex. Nature. 1994;367:654–657. [PubMed: 8107851]
- 11.
- Cao TB, Saier MH. The general protein secretory pathway: Phylogenetic analyses leading to evolutionary conclusions. Biochem Biophys Acta. 2003;1609:115–125. [PubMed: 12507766]
- 12.
- Pogliano JA, Beckwith J. SecD and SecF facilitate protein export in Escherichia coli. EMBO J. 1994;13:554–561. [PMC free article: PMC394844] [PubMed: 8313900]
- 13.
- Bakker EP, Randall LL. The requirement for energy during export of beta-lactamase in Escherichia coli is fulfilled by the total protonmotive force. EMBO J. 1984;3:895–900. [PMC free article: PMC557444] [PubMed: 6327294]
- 14.
- Brundage L, Henndrick JP, Schiebel E. et al. The purified E. coli integral membrane protein SecY/ E is sufficient for reconstitution of SecA-dependent precursor protein translocation. Cell. 1990;62:649–657. [PubMed: 2167176]
- 15.
- Duong F, Wickner W. Distinct catalytic roles of the SecYE, SecG and SecDFyajC subunits of preprotein translocase holoenzyme. EMBO J. 1997;16:2756–2768. [PMC free article: PMC1169885] [PubMed: 9184221]
- 16.
- Collinson I, Breyton C, Duong F. et al. Projection structure and oligomeric properties of a bacterial core protein translocase. EMBO J. 2001;20:2462–2471. [PMC free article: PMC125464] [PubMed: 11350935]
- 17.
- Breyton C, Haase W, Rapoport TA. et al. Three-dimensional structure of the bacterial protein-translocation complex SecYEG. Nature. 2002;418:662–665. [PubMed: 12167867]
- 18.
- Van den Berg B, Clemons JrWM, Collinson I. et al. X-ray structure of a protein-conducting channel. Nature. 2004;427:36–44. [PubMed: 14661030]
- 19.
- Pugsley AP. Translocation of proteins with signal sequences across membranes. Curr Opin Cell Biol. 1990;2:609–616. [PubMed: 2252587]
- 20.
- von HeijneG. Life and death of a signal peptide. Nature. 1998;396:111–113. [PubMed: 9823886]
- 21.
- Martoglio B, Dobberstein B. Signal sequences: More than just greasy peptides. Trends Cell Biol. 1998;8:410–415. [PubMed: 9789330]
- 22.
- Mothes W, Prehn S, Rapoport TA. Systematic probing of the environment of a translocating secretory protein during translocation through the ER membrane. EMBO J. 1994;13:3973–3982. [PMC free article: PMC395317] [PubMed: 8076593]
- 23.
- Plath K, Mothes W, Wilkinson BM. et al. Signal sequence recognition in posttranslational protein transport across the yeast ER membrane. Cell. 1998;94:795–807. [PubMed: 9753326]
- 24.
- Plath K, Wilkinson BM, Stirling CJ. et al. Interactions between Sec complex and prepro-alpha-factor during posttranslational protein transport into the endoplasmic reticulum. Mol Biol Cell. 2004;15:1–10. [PMC free article: PMC307522] [PubMed: 14617809]
- 25.
- Martoglio B, Hofmann MW, Brunner J. et al. The protein-conducting channel in the membrane of the endoplasmic reticulum is open laterally toward the lipid bilayer. Cell. 1995;81:207–214. [PubMed: 7736572]
- 26.
- Goder V, Junne T, Spiess M. Sec61p contributes to signal sequence orientation according to the positive-inside rule. Mol Biol Cell. 2004;15:1470–1478. [PMC free article: PMC363169] [PubMed: 14668483]
- 27.
- Goder V, Spiess M. Molecular mechanism of signal sequence orientation in the endoplasmic reticulum. EMBO J. 2003;22:3645–3653. [PMC free article: PMC165631] [PubMed: 12853479]
- 28.
- Simon SM, Blobel G. Signal peptides open protein-conducting channels in E coli. Cell. 1992;69:677–684. [PubMed: 1375130]
- 29.
- Harris CR, Silhavy TJ. Mapping an interface of SecY (PrlA) and SecE (PrlG) by using synthetic phenotypes and in vivo cross-linking. J Bacteriol. 1999;181:3438–3444. [PMC free article: PMC93811] [PubMed: 10348856]
- 30.
- Flower AM, Doebele RC, Silhavy TJ. PrlA and PrlG suppressors reduce the requirement for signal sequence recognition. J Bacteriol. 1994;176:5607–5614. [PMC free article: PMC196762] [PubMed: 8083155]
- 31.
- Derman AI, Puziss JW, Bassford Jr PJ. et al. A signal sequence is not required for protein export in prlA mutants of Escherichia coli. EMBO J. 1993;12:879–888. [PMC free article: PMC413286] [PubMed: 8458344]
- 32.
- Duong F, Wickner W. The PrlA and PrlG phenotypes are caused by a loosened association among the translocase SecYEG subunits. EMBO J. 1999;18:3263–3270. [PMC free article: PMC1171407] [PubMed: 10369667]
- 33.
- Sato K, Mori H, Yoshida M. et al. Short hydrophobic segments in the mature domain of ProOmpA determine its stepwise movement during translocation across the cytoplasmic membrane of Escherichia coli. J Biol Chem. 1997;272:5880–5886. [PubMed: 9038205]
- 34.
- Sato K, Mori H, Yoshida M. et al. In vitro analysis of the stop-transfer process during translocation across the cytoplasmic membrane of Escherichia coli. J Biol Chem. 1997;272:20082–20087. [PubMed: 9242681]
- 35.
- Duong F, Wickner W. Sec-dependent membrane protein biogenesis: SecYEG, preprotein hydrophobicity and translocation kinetics control the stop-transfer function. EMBO J. 1998;17:696–705. [PMC free article: PMC1170419] [PubMed: 9450995]
- 36.
- Saaf A, Wallin E, von HeijneG. Stop-transfer function of pseudo-random amino acid segments during translocation across prokaryotic and eukaryotic membranes. Eur J Biochem. 1998;251:821–829. [PubMed: 9490057]
- 37.
- Heinrich SU, Mothes W, Brunner J. et al. The Sec61p complex mediates the integration of a membrane protein by allowing lipid partitioning of the transmembrane domain. Cell. 2000;102:233–244. [PubMed: 10943843]
- 38.
- Beckmann R, Bubeck D, Grassucci R. et al. Alignment of conduits for the nascent polypeptide chain in the ribosome-Sec61 complex. Science. 1997;278:2123–2126. [PubMed: 9405348]
- 39.
- Ménétret JF, Neuhof A, Morgan DG. et al. The structure of ribosome-channel complexes engaged in protein translocation. Mol Cell. 2000;6:1219–1232. [PubMed: 11106759]
- 40.
- Meyer TH, Ménétret JF, Breitling R. et al. The bacterial SecY/E translocation complex forms channel-like structures similar to those of the eukaryotic Sec61p Complex. J Mol Biol. 1999;285:1789–1800. [PubMed: 9917412]
- 41.
- Manting EH, van Der Does C, Remigy H. et al. SecYEG assembles into a tetramer to form the active protein translocation channel. EMBO J. 2000;19:852–861. [PMC free article: PMC305625] [PubMed: 10698927]
- 42.
- Kaufmann A, Manting EH, Veenendaal AK. et al. Cysteine-directed cross-linking demonstrates that helix 3 of SecE is close to helix 2 of SecY and helix 3 of a neighboring secE. Biochemistry. 1999;38:9115–9125. [PubMed: 10413486]
- 43.
- Bessonneau P, Besson V, Collinson I. et al. The SecYEG preprotein translocation channel is a conformationally dynamic and dimeric structure. EMBO J. 2002;21:995–1003. [PMC free article: PMC125904] [PubMed: 11867527]
- 44.
- Veenendaal AK, Van Der Does C, Driessen AJ. The core of the bacterial translocase harbors a tilted transmembrane segment 3 of SecE. J Biol Chem. 2002;277:36640–36645. [PubMed: 12138117]
- 45.
- Clemons JrWM, Menetret JF, Akey CW. et al. Structural insight into the protein translocation channel. Curr Opin Struct Biol. 2004;14:390–396. [PubMed: 15313231]
- 46.
- Yahr TL, Wickner WT. Evaluating the oligomeric state of SecYEG in preprotein translocase. EMBO J. 2000;19:4393–4401. [PMC free article: PMC302025] [PubMed: 10944122]
- 47.
- Mori H, Tsukazaki T, Masui R. et al. Fluorescence resonance energy transfer analysis of protein translocase. SecYE from Thermus thermophilus HB8 forms a constitutive oligomer in membranes. J Biol Chem. 2003;278:14257–14264. [PubMed: 12533543]
- 48.
- Hanein D, Matlack KE, Jungnickel B. et al. Oligomeric rings of the Sec61p complex induced by ligands required for protein translocation. Cell. 1996;87:721–732. [PubMed: 8929540]
- 49.
- Hartl FU, Lecker S, Schiebel E. et al. The binding cascade of SecB to SecA to SecY/E mediates preprotein targeting to the E. coli plasma membrane. Cell. 1990;63:269–279. [PubMed: 2170023]
- 50.
- Economou A. Bacterial secretome: The assembly manual and operating instructions. Mol Membr Biol. 2002;19:159–169. [PubMed: 12463716]
- 51.
- Hunt JF, Weinkauf S, Henry L. et al. Nucleotide control of interdomain interactions in the conformational reaction cycle of SecA. Science. 2002;297:2018–2026. [PubMed: 12242434]
- 52.
- Caruthers JM, McKay DB. Helicase structure and mechanism. Curr Opin Struct Biol. 2002;12:123–133. [PubMed: 11839499]
- 53.
- Kimura E, Akita M, Matsuyama S. et al. Determination of a region in SecA that interacts with presecretory proteins in Escherichia coli. J Biol Chem. 1991;266:6600–6606. [PubMed: 1826108]
- 54.
- Baud C, Karamanou S, Sianidis G. et al. Allosteric communication between signal peptides and the SecA protein DEAD motor ATPase domain. J Biol Chem. 2002;277:13724–13731. [PubMed: 11825907]
- 55.
- Breukink E, Nouwen N, van Raalte A. et al. The C terminus of SecA is involved in both lipid binding and SecB binding. J Biol Chem. 1995;270:7902–7907. [PubMed: 7713885]
- 56.
- Fekkes P, de WitJG, Boorsma A. et al. Zinc stabilizes the SecB binding site of SecA. Biochemistry. 1999;38:5111–5116. [PubMed: 10213615]
- 57.
- Osborne AR, Clemons JrWM, Rapoport TA. A large conformational change of the translocation ATPase SecA. Proc Natl Acad Sci USA. 2004;101:10937–10942. [PMC free article: PMC491988] [PubMed: 15256599]
- 58.
- Snyders S, Ramamurthy V, Oliver D. Identification of a region of interaction between Escherichia coli SecA and SecY proteins. J Biol Chem. 1997;272:11302–11306. [PubMed: 9111035]
- 59.
- Matsumoto G, Yoshihisa T, Ito K. SecY and SecA interact to allow SecA insertion and protein translocation across the Escherichia coli plasma membrane. EMBO J. 1997;16:6384–6393. [PMC free article: PMC1170245] [PubMed: 9351821]
- 60.
- Mori H, Ito K. An essential amino acid residue in the protein translocation channel revealed by targeted random mutagenesis of SecY. Proc Natl Acad Sci USA. 2001;98:5128–5133. [PMC free article: PMC33175] [PubMed: 11309495]
- 61.
- Chiba K, Mori H, Ito K. Roles of the C-terminal end of SecY in protein translocation and viability of Escherichia coli. J Bacteriol. 2002;184:2243–2250. [PMC free article: PMC134956] [PubMed: 11914356]
- 62.
- Matsumoto G, Nakatogawa H, Mori H. et al. Genetic dissection of SecA: Suppressor mutations against the secY205 translocase defect. Genes Cells. 2000;5:991–999. [PubMed: 11168585]
- 63.
- Dapic V, Oliver D. Distinct membrane binding properties of N- and C-terminal domains of Escherichia coli SecA ATPase. J Biol Chem. 2000;275:25000–25007. [PubMed: 10835419]
- 64.
- Schiebel E, Driessen AJM, Hartl FU. et al. ΔμH+ and ATP function at different steps of the catalytic cycle of preprotein translocase. Cell. 1991;64:927–939. [PubMed: 1825804]
- 65.
- de KeyzerJ, van der Does C, Kloosterman TG. et al. Direct demonstration of ATP-dependent release of SecA from a translocating preprotein by surface plasmon resonance. J Biol Chem. 2003;278:29581–29586. [PubMed: 12771143]
- 66.
- Uchida K, Mori H, Mizushima S. Stepwise movement of preproteins in the process of translocation across the cytoplasmic membrane of Escherichia coli. J Biol Chem. 1995;270:30862–30868. [PubMed: 8537339]
- 67.
- van der Wolk JP, de WitJG, Driessen AJM. The catalytic cycle of the Escherichia coli SecA ATPase comprises two distinct preprotein translocation events. EMBO J. 1997;16:7297–7304. [PMC free article: PMC1170330] [PubMed: 9405359]
- 68.
- Economou A, Wickner W. SecA promotes preprotein translocation by undergoing ATP-driven cycles of membrane insertion and deinsertion. Cell. 1994;78:835–843. [PubMed: 8087850]
- 69.
- Eichler J, Wickner W. Both an N-terminal 65-kDa domain and a C-terminal 30-kDa domain of SecA cycle into the membrane at SecYEG during translocation. Proc Natl Acad Sci USA. 1997;94:5574–5581. [PMC free article: PMC20820] [PubMed: 9159114]
- 70.
- Kim YJ, Rajapandi T, Oliver D. SecA protein is exposed to the periplasmic surface of the E. coli inner membrane in its active state. Cell. 1994;78:845–853. [PubMed: 8087851]
- 71.
- van der Does C, den BlaauwenT, de Wit JG. et al. SecA is an intrinsic subunit of the Escherichia coli preprotein translocase and exposes its carboxyl terminus to the periplasm. Mol Microbiol. 1996;22:619–629. [PubMed: 8951810]
- 72.
- Ramamurthy V, Oliver D. Topology of the integral membrane form of Escherichia coli SecA protein reveals multiple periplasmically exposed regions and modulation by ATP binding. J Biol Chem. 1997;272:23239–23246. [PubMed: 9287332]
- 73.
- Sianidis G, Karamanou S, Vrontou E. et al. Cross-talk between catalytic and regulatory elements in a DEAD motor domain is essential for SecA function. EMBO J. 2001;20:961–970. [PMC free article: PMC145479] [PubMed: 11230120]
- 74.
- Fak JJ, Itkin A, Ciobanu DD. et al. Nucleotide exchange from the high-affinity ATP-binding site in SecA is the rate-limiting step in the ATPase cycle of the soluble enzyme and occurs through a specialized conformational state. Biochemistry. 2004;43:7307–7327. [PubMed: 15182175]
- 75.
- Woodbury RL, Hardy SJ, Randall LL. Complex behavior in solution of homodimeric SecA. Protein Sci. 2002;11:875–882. [PMC free article: PMC2373524] [PubMed: 11910030]
- 76.
- Ding H, Hunt JF, Mukerji I. et al. Bacillus subtilis SecA ATPase exists as an antiparallel dimer in solution. Biochemistry. 2003;42:8729–8738. [PubMed: 12873133]
- 77.
- Or E, Navon A, Rapoport T. Dissociation of the dimeric SecA ATPase during protein translocation across the bacterial membrane. EMBO J. 2002;21:4470–4479. [PMC free article: PMC126201] [PubMed: 12198149]
- 78.
- Driessen AJ. SecA, the peripheral subunit of the Escherichia coli precursor protein translocase, is functional as a dimer. Biochemistry. 1993;32:13190–13197. [PubMed: 8241173]
- 79.
- Benach J, Chou YT, Fak JJ. et al. Phospholipid-induced monomerization and signal-peptide-induced oligomerization of SecA. J Biol Chem. 2003;278:3628–3638. [PubMed: 12403785]
- 80.
- Duong F. Binding, activation and dissociation of the dimeric SecA ATPase at the dimeric SecYEG translocase. EMBO J. 2003;22:4375–4384. [PMC free article: PMC202361] [PubMed: 12941690]
- 81.
- Tziatzios C, Schubert D, Lotz M. et al. The bacterial protein-translocation complex: SecYEG dimers associate with one or two SecA molecules. J Mol Biol. 2004;340:513–524. [PubMed: 15210351]
- 82.
- Soultanas P, Wigley DB. DNA helicases: ‘Inching forward’ Curr Opin Struct Biol. 2000;10:124–128. [PubMed: 10679457]
- 83.
- Velankar SS, Soultanas P, Dillingham MS. et al. Crystal structures of complexes of PcrA DNA helicase with a DNA substrate indicate an inchworm mechanism. Cell. 1999;97:75–84. [PubMed: 10199404]
- 84.
- Driessen AJM. Precusor protein translocation by the Escherichia coli translocase is directed by the protonmotive force. EMBO J. 1992;11:847–853. [PMC free article: PMC556524] [PubMed: 1312464]
- 85.
- Geller BL, Green HM. Translocation of pro-OmpA across inner membrane vesicles of Escherichia coli occurs in two consecutive energetically distinct steps. J Biol Chem. 1989;264:16465–16469. [PubMed: 2674133]
- 86.
- Tani K, Shiozuka K, Tokuda H. et al. In vitro analysis of the process of translocation of OmpA across the Escherichia coli cytoplasmic membrane. A translocation intermediate accumulates transiently in the absence of the proton motive force. J Biol Chem. 1989;264:18582–18588. [PubMed: 2553715]
- 87.
- Driessen AJM, Wickner WT. Proton transfer is rate-limiting for translocation of precursor proteins by the Escherichia coli translocase. Proc Natl Acad Sci USA. 1991;88:2471–2475. [PMC free article: PMC51254] [PubMed: 1826054]
- 88.
- van DalenA, Killian A, de KruijffB. Delta-psi stimulates membrane translocation of the C-terminal part of a signal sequence. J Biol Chem. 1999;274:19913–19918. [PubMed: 10391938]
- 89.
- Daniels CJ, Bole DG, Quay SC. et al. Role for membrane potential in the secretion of protein into the periplasm of Escherichia coli. Proc Natl Acad Sci USA. 1981;78:5396–5400. [PMC free article: PMC348752] [PubMed: 7029533]
- 90.
- Nouwen N, de KruijffB, Tommassen J. PrlA suppressors in Escherichia coli relieve the proton electrochemical gradient dependency of translocation of wild-type precursors. Proc Natl Acad Sci USA. 1996;93:5953–5957. [PMC free article: PMC39169] [PubMed: 8650200]
- 91.
- Nishiyama KI, Fukuda A, Morita K. et al. Membrane deinsertion of SecA underlying proton motive force-dependent stimulation of protein translocation. EMBO J. 1999;18:1049–1058. [PMC free article: PMC1171196] [PubMed: 10022846]
- 92.
- Yamada H, Matsuyama S, Tokuda H. et al. A high concentration of SecA allows proton motive force-independent translocation of a model secretory protein into Escherichia coli membrane vesicles. J Biol Chem. 1989;264:18577–18581. [PubMed: 2553714]
- 93.
- van der Wolk JP, Fekkes P, Boorsma A. et al. PrlA4 prevents the rejection of signal sequence defective preproteins by stabilizing the SecA-SecY interaction during the initiation of translocation. EMBO J. 1998;17:3631–3639. [PMC free article: PMC1170699] [PubMed: 9649433]
- 94.
- Nishiyama K, Mizushima S, Tokuda H. A novel membrane protein involved in protein translocation across the cytoplasmic membrane of Escherichia coli. EMBO J. 1993;12:3409–3415. [PMC free article: PMC413615] [PubMed: 8253068]
- 95.
- Nishiyama K, Hanada M, Tokuda H. Disruption of the gene encoding p12 (SecG) reveals the direct involvement and important function of SecG in the protein translocation of Escherichia coli at low temperature. EMBO J. 1994;13:3272–3277. [PMC free article: PMC395223] [PubMed: 8045257]
- 96.
- Matsumoto G, Mori H, Ito K. Roles of SecG in ATP- and SecA-dependent protein translocation. Proc Natl Acad Sci USA. 1998;95:13567–13572. [PMC free article: PMC24859] [PubMed: 9811840]
- 97.
- Hanada M, Nishiyama K, Tokuda H. SecG plays a critical role in protein translocation in the absence of the proton motive force as well as at low temperature. FEBS Lett. 1996;381:25–28. [PubMed: 8641431]
- 98.
- Nishiyama K, Suzuki T, Tokuda H. Inversion of the membrane topology of SecG coupled with SecA-dependent preprotein translocation. Cell. 1996;85:71–81. [PubMed: 8620539]
- 99.
- Nouwen N, Driessen AJM. SecDFyajC forms a heterotetrameric complex with YidC. Mol Microbiol. 2002;44:1397–1405. [PubMed: 12068816]
- 100.
- Pogliano KJ, Beckwith J. Genetic and molecular characterization of the Escherichia coli secD operon and its products. J Bacteriol. 1994;176:804–814. [PMC free article: PMC205118] [PubMed: 7507921]
- 101.
- Bolhuis A, Broekhuizen CP, Sorokin A. et al. SecDF of Bacillus subtilis, a molecular Siamese twin required for the efficient secretion of proteins. J Biol Chem. 1998;273:21217–21224. [PubMed: 9694879]
- 102.
- Kato Y, Nishiyama K, Tokuda H. Depletion of SecDF-YajC causes a decrease in the level of SecG: Implication for their functional interaction. FEBS Lett. 2003;550:114–118. [PubMed: 12935896]
- 103.
- Duong F, Wickner W. The SecDFyajC domain of preprotein translocase controls preprotein movement by regulating SecA membrane cycling. EMBO J. 1997;16:4871–4879. [PMC free article: PMC1170122] [PubMed: 9305629]
- 104.
- Economou A, Pogliano JA, Beckwith J. SecA membrane cycling at SecYEG is driven by distinct ATP binding and hydrolysis events and is regulated by SecD and SecF. Cell. 1995;83:1171–1181. [PubMed: 8548804]
- 105.
- Matsuyama S, Fujita Y, Mizushima S. SecD is involved in the release of translocated secretory proteins from the cytoplasmic membrane of Escherichia coli. EMBO J. 1993;12:265–270. [PMC free article: PMC413201] [PubMed: 8428584]
- Introduction
- The SecYEG Translocon at the Atomic Level
- Binding and Orientation of the Leader Peptide into the Translocon
- Opening of the Translocation Channel
- Translocation Pause
- The Quaternary Structure of the SecYEG Translocon
- Dynamic Behavior of SecYEG Oligomers
- Atomic Structure of the SecA Translocation Motor
- Binding of the SecA Motor to the SecYEG Channel
- How Does SecA Use ATP to Catalyze Translocation?
- The SecA Monomer-Dimer Equilibrium
- The Translocase Makes Use of the Proton Motive Force
- Additional Subunits Make the Translocase Holo-Enzyme
- Concluding Remarks
- Acknowledgments
- References
- Preprotein Translocation through the Sec Translocon in Bacteria - Madame Curie B...Preprotein Translocation through the Sec Translocon in Bacteria - Madame Curie Bioscience Database
- Functional Evidence of Pain Control by the Immune System - Madame Curie Bioscien...Functional Evidence of Pain Control by the Immune System - Madame Curie Bioscience Database
- Future Perspectives of Interstitial and Perfusional Hyperthermia - Madame Curie ...Future Perspectives of Interstitial and Perfusional Hyperthermia - Madame Curie Bioscience Database
- α1-Microglobulin - Madame Curie Bioscience Databaseα1-Microglobulin - Madame Curie Bioscience Database
- Macroautophagy in Mammalian Cells - Madame Curie Bioscience DatabaseMacroautophagy in Mammalian Cells - Madame Curie Bioscience Database
Your browsing activity is empty.
Activity recording is turned off.
See more...