NCBI Bookshelf. A service of the National Library of Medicine, National Institutes of Health.
Madame Curie Bioscience Database [Internet]. Austin (TX): Landes Bioscience; 2000-2013.
Summary
Transfer-messenger RNA (tmRNA), also known as SsrA or 10Sa RNA, is a bacterial ribonucleic acid that recycles 70S ribosomes stalled on problematic messenger RNAs (mRNAs) and also contributes to the degradation of incompletely synthesized peptides. tmRNA acts initially as a transfer RNA (tRNA), being aminoacylated at its 3' end by alanyl-tRNA synthetase, to add alanine to the polypeptide chain. Translation then resumes not on the mRNA on which the ribosomes were stalled but at an internal position in tmRNA. Termination soon occurs, tmRNA recruiting the appropriate termination factors allowing the release of the tagged protein that is subsequently recognized and degraded by specific proteases. As a consequence, the ribosome can be recycled. Moreover, the tRNA function of tmRNA can be utilized by some bacteriophages as an important component for sensing the overall state of the host cell to control prophage repression. This chapter focuses on our current understanding of tmRNA structure and function, with an emphasis on its recognition by a class II aminoacyl-tRNA synthetase.
Introduction
Protein synthesis is a central component of all living cells. Recent structural information collected on intact ribosomes or isolated subunits from bacteria and archea is revolutionizing our understanding of protein synthesis (for a review, see ref. 1). While protein synthesis is a highly accurate and tightly regulated process, problems can still arise during messenger RNA (mRNA) decoding, and have to be dealt with quickly and efficiently. In bacteria, problematic mRNAs lacking a termination codon can be produced due to mRNA degradation and/or transcription drop off, hence stalling and sequestring translating ribosomes. Incomplete polypeptides and depleted levels of free ribosomes can be harmful for the cell, especially during physical or chemical stresses. In bacteria and some chloroplasts, a special RNA molecule, transfer-message RNA (tmRNA, SsrA or 10Sa RNA), rescues stalled ribosomes and contributes to the degradation of incompletely synthesized peptides. This molecule is unique, acting both as a transfer RNA and as a messenger RNA to orchestrate an unusual reaction referred to as trans-translation (for recent reviews, see refs. 2, 3).
Figure 1 illustrates our current understanding of tmRNA function in bacteria (most of the available data are from E. coli). In prokaryotes, translation can initiate on mRNA that lacks a termination codon and continue until the ribosome reaches the last readable codon. This ribosome stalls and also blocks the progress of any other elongating ribosomes on the same message. tmRNA prevents ribosome stalling, first acting as a transfer RNA, being aminoacylated at its 3' end with alanine by alanyl-tRNA synthetase (AlaRS)4,5 and adds alanine to the stalled polypeptide chain. Resumption of translation ensures not on the mRNA on which the ribosomes were stalled but at an internal position in tmRNA (the resume codon). Since tmRNA internal Open Reading Frame (ORF) harbors a termination codon, termination soon occurs and permits ribosome recycling. Also, the degradation tag encoded by the sequence in tmRNA, then helps to ensure intracellular proteolytic specificity. In eukaryotes, problematic proteins are targeted to the 26S proteasome by posttranslational addition of polyubiquitin. In bacteria, proteins bearing the tmRNA-encoded tag are recognized by several different proteases (fig. 1B). ClpXP, ClpAP and FtsH are multicomponent enzymes that promote ATP-dependent degradation of the tmRNA-tagged proteins in the bacterial cytoplasm (ClpX has a specificity-enhancing factor, SspB), while tmRNA-tagged proteins with signal sequences are exported to the periplasmic compartment where they are degraded by the Tsp protease.
tmRNA was initially shown to ensure the degradation of proteins produced by incomplete translation.6 Tagging is also observed when ribosomes stall at internal sites on complete messages,7 while the ribosome is at the termination codon8 and “readthrough” of canonical termination codons (fig. 1A). In the last case, the tag is added after some extension of the polypeptide past the stop codon (H. Aiba, personal communication). This mechanism in bacteria and some chloroplasts ensures intracellular protein quality control and that the stalled ribosomes are recycled.
In E. coli cells, several endogenous mRNA targets for tmRNA-mediated trans-translation have been recently identified. These are messages encoding LacI (tagging of LacI was dependent on its binding to its operators)9 and λ cI repressors, YbeL, GalE and RbsK,8 as well as many other unidentified proteins that are expressed at low levels. Thus, some proteins involved in sugar metabolism (LacI, GalE and RbsK) and during phage infections (λ cI) are subjected to tagging by tmRNA. During infection by Salmonella enterica (serovar Typhimurium), tmRNA is required for full virulence and either enhances (vacB, asnS, thiI) or represses (f304, dedE, yoaA) the expression of specific genes during infection.10
Transcription and Maturation of tmRNA
In Escherichia coli, the 457 nucleotide (nt) tmRNA precursor is encoded by a monocistronic gene controlled by a σ70-type promoter and ending in a rho-independent transcriptional terminator. 11 Transforming precursor tmRNA into its mature form is a complex process that involves several enzymes (fig. 2). The fully mature 363 nt E .coli tmRNA results from cleavage of the precursor by a number of RNases. RNase P generates the 5'end of mature tmRNA as it does for canonical tRNAs.4 Trimming at the 3' end involves ribonuclease T and PH.12 Moreover, ribonuclease E cuts the RNA precursor at three locations near its 3' end to generate a 3'-CCA that can be aminoacylated by alanine.13 Two post-transcriptional modifications, 5-methyluridine and pseudouridine, are present in the tRNA-like portion of E. coli tmRNA, within a 7-nt loop that mimicks the conserved TΨC-loops of canonical tRNAs (fig. 3A).14 Purified tRNA(m5U54)-methyltransferase (RUMT)15 and purified tRNA psi 55 synthase16 recognize and modify U341 and U342 from a synthetic tmRNA in vitro, respectively, as demonstrated by combined liquid chromatography/electrospray ionization mass spectrometry (Felden B and Crain PF, unpublished results) suggesting these enzymes might be responsible in vivo. Recent genomic data for Clostridium botulinum has revealed a group I intron occurring before the last 3'-nucleotide of the tmRNA T-loop.17 The intron is small (287 nucleotides), contains no ORF, and possesses the canonical primary and secondary structural features of group I introns, including many self-splicing RNAs. The pre-tmRNA is expected to be spliced in vivo (fig.2), to yield a functional tmRNA molecule.
tmRNA Phylogeny
tmRNA is ubiquitous in eubacteria and some chloroplasts. Also, a degenerated tmRNA gene homologue was recovered in a mitochondria, but lacking a tag reading frame. tmRNA has been searched by PCR in ten representative archaeal genomes of archeas and has not been detected (Felden B, unpublished data). The number of known tmRNA sequences has doubled in the last year, due to both the rapid completion of many bacterial genome projects, and to the ease with which tmRNA sequences can be amplified by PCR thanks to its high degree of sequence conservation at both 5' and 3'-ends.18-22 As of October 2001, 230 sequences are available from 197 species (41 uncultured) of 15 phyla.17 Phylogenetic studies of all these sequences have refined the tmRNA secondary structure predictions and led to the first proposals for tertiary interactions outside of the tRNA-like domain.20-21 tmRNA secondary structure contains a perfect analog of the acceptor branch of tRNAAla, several RNA helices and usually four pseudoknots (fig. 3). Among all the pseudoknots, only PK1 is required for tmRNA function in E. coli, while the other three (PK2-PK4) are interchangeable and can be substituted with single-stranded RNAs.23 Circularly permuted versions of tmRNA have been found in alpha proteobacteria and a lineage of cyanobacteria.24 The tmRNA in each species arose from an independent permutation event. As a result of the altered genetic structure, these tmRNAs are composed of two distinct RNAs.
The tRNA-Like Structure of tmRNA
In all the available tmRNA sequences, the 5' end pairs with the 3' end to form a tRNA-acceptor stem with a 3'-CCA sequence. The tRNA-like structure also includes a TΨC stemloop.4-5 Probing data acquired in solution25 together with the presence and location of two tRNA-specific post-transcriptional modifications within a seven nucleotide T-loop14 have reinforced the overall structural analogy of part of E. coli tmRNA with canonical tRNAs (red nucleotides in fig.3). Recent studies based on (i) modeling and transient electric birefringence,26 (ii) the identification of UV cross-linked sites within the tRNA-like region of tmRNA27 and (iii) an extensive mutational analysis of the conserved nucleotides around the tRNA-like structure of tmRNA28 suggest that its structural mimicry with canonical tRNAs extends beyond the acceptor branch (fig. 4). Part of a long disrupted stem (H5), capped with a D loop like consensus sequence 5'-N3-5GGN1-3UYGA-3' is proposed to be analogous to the anticodon stem of canonical tRNAs. There is no phylogenetic or structural (probing in solution) evidences supporting the presence of a D-stem in bacterial tmRNAs. The angle between the tmRNA acceptor branch (H1-H6, fig. 4) and stem H5 is proposed to be 111°-137°, larger than between the acceptor and the anticodon branches in canonical tRNAs.26 In the absence of ligands, the tRNA-like domain of tmRNA might be more flexible than canonical tRNAs, probably lacking of some of the tertiary interactions found in canonical tRNAs. X-ray studies of tmRNA or NMR studies of its isolated tRNA-like fragment, will provide a definitive and accurate answer about the extent of the structural mimicry of tmRNA with canonical tRNAs which is, until now, still unclear.
Aminoacylation of tmRNA
One of tmRNA's characteristics is that it can be specifically recognized and charged by an aminoacyl-tRNA synthetase, AlaRS. Despite being ∼5 times larger than tRNA alanine, E. coli tmRNA is specifically aminoacylated by E. coli alanyl-tRNA synthetase in vitro (Table 1). Recognition of tRNAAla by E. coli alanyl-tRNA synthetase (AlaRS) depends largely on a single G3·U70 base pair in the acceptor stem. Introduction of this base pair into other tRNAs confers alanine acceptance upon them.29-30 A G·U wobble base pair at the third position of the acceptor stem and an adenosine at the discriminator position (the position adjacent to the 3'-terminal CCA) are both responsible for aminoacylation of tmRNA (fig. 3A). Both sites are conserved in all the available tmRNA sequences from prokaryotes and chloroplasts. Replacing the G·U pair by either G·A or G·C prevents aminoacylation of tmRNA with alanine. In contrast, in vivo data suggest that a mutant tmRNA with a G·C pair instead of a G·U pair hardly functions at all, whereas the G·A mutant is partially functional,4 which raises the question of how essential the GU pair really is.
Table 1
Kinetics of aminoacylation of native and synthetic tmRNA compared with native and synthetic tRNAAla.
The aminoacylation plateau for native purified tRNAAla is ∼100%. In contrast, synthetic or native purified tmRNA can only be aminoacylated by purified AlaRS to ∼20-40% in vitro (Table 1). This suggests that the solution conformation of tmRNA on its own is not optimal for aminoacylation, perhaps because of excessive conformational flexibility, and might require a trans-acting factor to be fully aminoacylated. Probing the solution conformation of E. coli tmRNA in vitro, in the absence of ligands, have shown that some structural domains of the RNA are unstable and adopt several conformations.25 Alternatively, a significant fraction of tmRNA might have to be uncharged in vivo, perhaps to prevent its competition with EF-Tu-GTP/Ala-tRNAAla for ribosomal A-site binding. As well as differences in plateau levels, there are also differences in the rates of aminoacylation between tmRNA and tRNA. Synthetic tmRNA is alanylated by AlaRS at a ∼5-fold lower rate (kcat) and a ∼16-fold decreased affinity (KM) compared to synthetic tRNAAla (Table 1), perhaps partly because of the latter argument. Moreover, since E. coli tmRNA is five times larger than tRNAAla, it likely accounts for some of the differences between tmRNA and tRNAAla aminoacylation by AlaRS. A direct comparison of the kinetic parameters between native tmRNA and native tRNAAla is not yet available. Based on a set of full-length deoxynucleotide substituted tRNAAla, there are backbone interactions with alanyl-tRNA synthetase in both the acceptor stem and the T-loop.31 The structural basis of the interaction between AlaRS and tmRNA, however, is unknown.
By transplanting the upper half of the acceptor stem of tRNAHis into E. coli tmRNA, the variant switches from alanine to histidine acceptance, but more importantly this histidine acceptor tmRNA variant retains the ability of incorporating tag-specific amino acids into the polypeptide in vitro.32 Histidine, which does not belong to the original tag-peptide, is incorporated into the mutant-directed tag. These results indicate that the first residue of the tag-peptide is brought by tmRNA and is substitutable by another amino acid.
Recruitment of Alanyl-tmRNA by the 70S Ribosome
During translation in prokaryotes, elongation Factor Tu (EF-Tu) delivers most of the aminoacyl-tRNAs in complex with GTP to the ribosome, protecting the aminoacyl moiety and preventing deacylation. EF-Tu interacts with tmRNA in vitro33-34 and probably also in vivo (Table 2 and fig. 5). The association constant of synthetic Ala-tmRNA with his-tagged EF-Tu.GTP is ∼150-fold lower than for synthetic Ala-tRNAAla. This lower affinity of EF-Tu for Ala-tmRNA may be important to ensure tmRNA does not perturb or compete with Ala-tRNA during regular protein synthesis. The affinity of tmRNA may have been tuned in a similar way to that of misacylated tRNA.35 An unusual interaction of deacylated tmRNA with either EF-Tu.GDP or EF-Tu.GTP has been described recently.36 Its physiological relevance, however, remains to be established.
Table 2
tmRNA-associated ligands in E. coli.
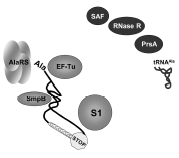
Figure 5
The ligands of tmRNA. Refer to the text and Table 2 for a description of their functions.
Another protein, small protein B (SmpB), binds specifically and with high affinity to tmRNA in vitro and is required for tmRNA-mediated peptide tagging and stable association of tmRNA with ribosomes in vivo (Table 1 and fig. 5).37 Gene disruption of SmpB is equivalent to that observed when disrupting SsrA.37 Ribosomal protein S1 is also required for tmRNA binding to isolated and poly U-programmed ribosomes.38 S1 is attached to the 30S subunit via its N-terminal moiety. Its direct visualization by cryo-electron microscopy has confirmed its involvement in mRNA binding.39 Nucleotides that are cross-linked between tmRNA and S1 are located before and within the coding sequence, as well as within two of the four pseudoknots in E. coli tmRNA, PK2 and PK3.38 The RNA-unwinding activity of S1 could facilitate the function of tmRNA by unfolding some of the intricate RNA structures surrounding the resume codon. Thus, tmRNA internal ORF will become more accessible to the translational apparatus during re-registration and translation. Interestingly, ribosomal protein S1 is not found in all bacterial lineage's, especially the low G+C group of Gram-positive bacteria. One exception is in B. subtilis, where a gene encoding a protein highly homologous to S1 has been identified.40 Hence, at least in some species of the low G+C group, tmRNA might have to function without S1.
Additional Ligands of tmRNA
Several additional proteins are associated with tmRNA in vivo (Table 2), when both tmRNA and SmpB are overproduced.41 Their implications during trans-translation remain elusive. Among them, genetic evidences reinforce the functional link between tmRNA and phosphoribosyl pyrophosphate synthase.42-43 In Salmonella enterica (Serovar Typhimurium), the expression of RNase R, encoded by vacB, was initially identified as a gene induced by ssrA.10 Interestingly, the encoded protein, a member of the RNase II family, is also associated with tmRNA in E. coli cells, and might be involved in degrading the problematic message during trans-translation. Aminoacylatable RNAs can also bind tmRNA with specificity in vitro.44 E. coli tmRNA interacts specifically with native E. coli tRNA alanine (tRNAAla), alanine being the first codon of tmRNA internal open reading frame. Interestingly, the most abundant tRNAAla isoacceptor in vivo, which is the only one that is able to form three canonical pairs with the resume codon, binds tmRNA with the highest affinity (a 0.5 μM Kd). AlaRS could take advantage of the proximity of tmRNA and tRNAAla during aminoacylation. Complex formation between tmRNA and tRNAAla, however, awaits to be recovered in vivo to confirm a possible relevance to tmRNA function in the bacterial cell. tmRNA also modulates the function of several DNA binding proteins (LacI, LexA and phage P22 C1 transcription activator) by binding them and reducing their free concentration in the cell.45
Importance of ssrA and smpB for Survival and Pathogenesis
Trans-translation occurs quite frequently in bacterial cells. However, depending on the strains tmRNA might or might not be lethal.3 In E. coli, ssrA or smpB are not essential for growth but minor phenotypes are associated with disrupting either ssrA or smpB: compared to wild-type, mutant cells recover from carbon starvation at a slower rate, exhibit a reduced mobility in soft agar, a slower growth rate at 42°C and an inability to support the growth of some bacteriophages.4,37,46 Disruption of ssrA in Bacillus subtilis leads to growth defects under physical and chemical stresses such as elevated temperatures or the presence of ethanol or cadmium chloride.47 In Neisseria gonorrhoeae, tmRNA-directed rescue of stalled protein synthesis preventing depletion of free ribosomes is essential for viability, whereas tagging for proteolysis is dispensable48. In another pathogen, Salmonella enterica (serovar Typhimurium), tmRNA is also required for full virulence.10
tmRNA and Bacteriophages
Some bacterial tmRNAs serve as the insertion site for acquired sequences such as phages49 and pathogenicity islands.50 In addition it can also play a role in phage life cycle. For example in E. coli, tmRNA is required for the growth of λimmP22.51 Protein tagging is essential for λimmP22 growth in E. coli, the degradation of the tagged proteins being necessary to achieve optimal levels of phage growth.52 SsrA- strains infected by bacteriophage Mu accumulate truncated forms of repressor Repc, a key regulator of the phage reproductive cycle.53 Repc is directly responsible for Mu prophage repression by binding to a promoter consisting of nine repressor-binding motifs. Truncated forms of Repc are able to bind the promoter with a higher affinity, even at higher temperatures. As a consequence, phage mutants that are normally thermoinducible loose this capacity. tmRNA is essential during the process and appears to be used by some bacteriophages as an important component for sensing the overall state of the host cell, controlling prophage repression, hence influencing the lysis-lysogeny decision.
Molecular Origins of tmRNA Sequences
Since bacterial tmRNAs serve as the insertion site for acquired sequences, it has probably contributed to the sequence diversity and variability observed today. In all living organisms, mRNAs carry the genetic information and aminoacyl-tRNAs deliver the aminoacids to the ribonucleoprotein factory, the ribosome, in which peptide bond formation and decoding take place. With tmRNA, a tRNA-like structure and a short translatable internal open reading frame are combined into a single molecule. This interesting and unique feature of tmRNA suggests that it may have resulted from the fusion of two genes, one encoding a tRNA, another a mRNA. Conversely, tmRNA might have been the progenitor of some of the bacterial messenger RNAs and transfer RNAAla that are present today, perhaps as a molecular remnant during the evolution of the genetic code. Today's sophisticated translational apparatus has probably evolved from a much simpler version, in which RNAs were the only players. Among them, tRNAs and especially tRNA-like structures are probably among the most significant relics of this RNA world, since they are ubiquitous, their structures is highly conserved and they are responsible for various functions in cellular metabolism.54 Moreover, tRNA mimics are also involved in biological processes other than ribosome-dependent translation.55
tRNA-like structures have arisen early on during genomic evolution and played essential roles in the earliest replicating systems, before the advent of protein synthesis.56 The tRNA-like structure of tmRNA might originate from these primitive roles of tRNA-like structures in replication. Although tmRNA has no canonical anticodon arm, part of tmRNA structure has analogy with a tRNAAla acceptor branch (fig. 3). Top (acceptor/T stem-loops) and bottom (anticodon/D stem-loops) halves of tRNAs are structurally and functionally independent units and could have evolved independently. Thus, molecular evolution might have maintained tmRNA's tRNA-like structure for functional requirements, while the bottom half might have evolved on its own. In the case of tmRNA the “bottom half ” is, for the most part, made of hundreds of nucleotides folded into several RNA pseudoknots. Stretches of RNA pseudoknots are commonly found within the 3'-untranslated regions of plant viral genomic RNAs possessing a partial tRNA-like structure at their 3'-ends, the most famous example being the 3'-end of tobacco mosaic virus RNA. Since tmRNA serves as the attached site for some bacteriophages, one hypothesis might be that tmRNA arose from the invasion of a tRNAAla gene by a viral RNA.
Concluding Remarks
Since the initial proposal of a tmRNA-mediated peptide tagging system by the Sauer's lab six years ago, a variety of biochemical, genetic, phylogenetic and structural data have accumulated, providing evidences of how this remarkable ribonucleic acid might act in bacteria. The detailed mechanisms underlying tmRNA-directed protein tagging in bacteria are, however, poorly understood. Specific recognition and aminoacylation of tmRNA by alanyl-tRNA synthetase is involved in the early steps of trans-translation. Expression of tmRNA is important for survival, growth under stress, and bacterial pathogenesis. Several endogenous mRNA targets for tmRNA-mediated trans-translation have been identified recently. Specific ligands of tmRNA have been recovered, including proteins and RNAs. tmRNA tags proteins and recycles stalled ribosomes in a variety of instances, and tmRNA-mediated trans-translation is able to compete against either readthrough or translation termination at canonical termination codons (J. Collier and P. Bouloc, personal communication), suggesting a broader implication of tmRNA in bacterial physiology than originally thought.
Addendum
Recent data from three independent groups showed that proteins SmpB (Hanawa-Suetsugu et al. Nucleic Acids Res 2002 30:1620-1629; Shimizu and Ueda, FEBS Lett 2002; 514:74-77) and EF-Tu.GTP (Barends et al. J Mol Biol 2001; 314:9-21) enhances alanine-accepting activity of tmRMA; also, there is an additive and even a weak synergistic effect of their combined presence, relative to their individual stimulation.
Acknowledgments
We thank Dr SC. Harvey for providing us with the model of tmRNA's tRNA-like part, Drs H. Aiba and P. Bouloc for access to results prior to publication and Dr A. Carter for critical reading of the manuscript. This work was funded by a Human Frontier Science Program Research Grant (RG0291/2000-M 100), by a Research Grant entitled “Recherche Fondamentale en Microbiologie et maladies infectieuses” from the “Institut Pasteur” and by an “Action Concertée Incitative Jeunes Chercheurs 2000” from the French Ministry of Research, to B.F.
References
- 1.
- Ramakrishnan V, Moore PB. Atomic structures at last: the ribosome in 2000. Curr Opin Struct Biol. 2001;11:144–154. [PubMed: 11297922]
- 2.
- Karzai AW, Roche ED, Sauer RT. The SsrA-SmpB system for protein tagging, directed degradation and ribosome rescue. Nat Struct Biol. 2000;7:449–455. [PubMed: 10881189]
- 3.
- Gillet R, Felden B. Emerging views on tmRNA mediated protein tagging and ribosome rescue. Mol Microbiol. 2001;42:879–885. [PubMed: 11737633]
- 4.
- Komine Y, Kitabatake M, Yokogawa T. et al. A tRNA-like structure is present in 10Sa RNA, a small stable RNA from Escherichia coli. Proc Natl Acad Sci USA. 1994;91:9223–9227. [PMC free article: PMC44784] [PubMed: 7524073]
- 5.
- Ushida C, Himeno H, Watanabe T. et al. tRNA-like structures in 10Sa RNAs of Mycoplasma capricolum and Bacillus subtulis. Nucl Acids Res. 1994;22:3392–3396. [PMC free article: PMC523734] [PubMed: 7521527]
- 6.
- Keiler KC, Waller PR, Sauer RT. Role of a peptide tagging system in degradation of proteins synthesized from damaged messenger RNA. Science. 1996;271:990–993. [PubMed: 8584937]
- 7.
- Roche ED, Sauer RT. Ssra-mediated peptide tagging caused by rare codons and tRNA scarcity. EMBO J. 1999;18:4579–4589. [PMC free article: PMC1171532] [PubMed: 10449423]
- 8.
- Roche ED, Sauer RT. Identification of endogenous SsrA-tagged proteins reveals tagging at positions corresponding to stop codons. J Biol Chem. 2001;276:28509–28515. [PubMed: 11373298]
- 9.
- Abo T, Inada T, Ogawa K. et al. SsrA-mediated tagging and proteolysis of LacI and its role in the regulation of lac operon. EMBO J. 2000;19:3762–769. [PMC free article: PMC313975] [PubMed: 10899129]
- 10.
- Julio SM, Heithoff DM, Mahan MJ. ssrA (tmRNA) plays a role in Salmonella enterica serovar Typhimurium pathogenesis. J Bacteriol. 2000;182:1558–1563. [PMC free article: PMC94452] [PubMed: 10692360]
- 11.
- Chauhan AK, Apirion D. The gene for a small stable RNA (10SA RNA) of Escherichia coli. Mol Microbiol. 1989;3:1481–1485. [PubMed: 2482406]
- 12.
- Li Z, Pandit S, Deutscher MP. 3' exoribonucleolytic trimming is a common feature of the maturation of small, stable RNAs in Escherichia coli. Proc Natl Acad Sci USA. 1998;95:2856–2861. [PMC free article: PMC19659] [PubMed: 9501180]
- 13.
- Lin-Chao S, Wei CL, Lin YT. RNase E is required for the maturation of ssrA RNA and normal ssrA RNA peptide-tagging activity. Proc Natl Acad Sci USA. 1999;96:12406–12411. [PMC free article: PMC22933] [PubMed: 10535935]
- 14.
- Felden B, Hanawa K, Atkins JF. et al. Presence and location of modified nucleotides in Escherichia coli tmRNA: structural mimicry with tRNA acceptor branches. EMBO J. 1998;17:3188–3196. [PMC free article: PMC1170657] [PubMed: 9606200]
- 15.
- Kealey JT, Santi DV. High-level expression and rapid purification of tRNA (m5U54)-methyltransferase. Protein Expr Purif. 1994;5:149–152. [PubMed: 8054847]
- 16.
- Nurse K, Wrzesinski J, Bakin A. et al. Purification, cloning, and properties of the tRNA psi 55 synthase from Escherichia coli. RNA. 1995;1:102–112. [PMC free article: PMC1369054] [PubMed: 7489483]
- 17.
- Williams KP. The tmRNA website: invasion by an intron Nucl Acids Res 200230in press . [PMC free article: PMC99078] [PubMed: 11752287]
- 18.
- Williams KP, Bartel DP. Phylogenetic analysis of tmRNA secondary structure. RNA. 1996;2:1306–1310. [PMC free article: PMC1369456] [PubMed: 8972778]
- 19.
- Felden B, Gesteland RF, Atkins JF. Eubacterial tmRNAs: everywhere except the alpha-proteobacteria? Biochim Biophys Acta. 1999;1446:145–148. [PubMed: 10395928]
- 20.
- Felden B, Massire C, Westhof E. et al. Phylogenetic analysis of tmRNA genes within a bacterial subgroup reveals a specific structural signature. Nucleic Acids Res. 2001;29:1602–1607. [PMC free article: PMC31281] [PubMed: 11266563]
- 21.
- Kelley ST, Harris JK, Pace NR. Evaluation and refinement of tmRNA structure using gene sequences from natural microbial communities. RNA. 2001;9:1310–1306. [PMC free article: PMC1370174] [PubMed: 11565752]
- 22.
- Schonhuber W, Bourhis GL, Tremblay J. Utilization of tmRNA sequences for bacterial identification BMC Microbiol. 2001. p. 20. [PMC free article: PMC55692] [PubMed: 11560762]
- 23.
- Nameki N, Tadaki T, Himeno H. et al. Three of four pseudoknots in tmRNA are interchangeable and are substitutable with single-stranded RNAs. FEBS Lett. 2000;470:345–349. [PubMed: 10745094]
- 24.
- Keiler KC, Shapiro L, Williams KP. tmRNAs that encode proteolysis-inducing tags are found in all known bacterial genomes : a two-piece tmRNA functions in Caulobacter. Proc Natl Acad Sci USA. 2000;97:7778–7783. [PMC free article: PMC16621] [PubMed: 10884408]
- 25.
- Felden B, Himeno H, Muto A. et al. Probing the structure of the Escherichia coli 10Sa RNA (tmRNA). RNA. 1997;3:89–103. [PMC free article: PMC1369465] [PubMed: 8990402]
- 26.
- Stagg SM, Frazer-Abel AA, Hagerman PJ. et al. Structural studies of the tRNA domain of tmRNA. J Mol Biol. 2001;309:727–735. [PubMed: 11397092]
- 27.
- Zwieb C, Guven SA, Wower IK. et al. Three-Dimensional Folding of the tRNA-like Domain of Escherichia coli tmRNA. Biochemistry. 2001;40:9587–9595. [PubMed: 11583158]
- 28.
- Hanawa-Suetsugu K, Bordeau V, Himeno H. et al. Importance of the conserved nucleotides around the tRNA-like structure of Escherichia coli transfer-messenger RNA for protein tagging Nucleic Acid Res 2001. in press . [PMC free article: PMC92554] [PubMed: 11713316]
- 29.
- Hou YM, Schimmel P. A simple structural feature is a major determinant of the identity of a transfer RNA. Nature. 1988;333:140–145. [PubMed: 3285220]
- 30.
- McClain WH, Foss K. Changing the acceptor identity of a transfer RNA by altering nucleotides in a ‘variable pocket’ Science. 1988;241:1804–1807. [PubMed: 2459773]
- 31.
- Pleiss JA, Wolfson AD, Uhlenbeck OC. Mapping contacts between Escherichia coli Alanyl tRNA Synthetase and 2' hydroxyls using a complete tRNA molecule. Biochemistry. 2000;39:8250–8258. [PubMed: 10889033]
- 32.
- Nameki N, Tadaki T, Muto A. et al. Amino acid acceptor identity switch of Escherichia coli tmRNA from alanine to histidine in vitro. J Mol Biol. 1999;289:1–7. [PubMed: 10339400]
- 33.
- Rudinger-Thirion J, Giege R, Felden B. Aminoacylated tmRNA from Escherichia coli interacts with prokaryotic elongation factor Tu. RNA. 1999;5:989–992. [PMC free article: PMC1369822] [PubMed: 10445873]
- 34.
- Barends S, Wower J, Kraal B. Kinetic parameters for tmRNA binding to alanyl-tRNA synthetase and Elongation Factor Tu from Escherichia coli. Biochemistry. 2000;39:2652–2658. [PubMed: 10704215]
- 35.
- LaRiviere FJ, Wolfson AD, Uhlenbeck OC. Uniform binding of aminoacyl-tRNAs to elongation factor Tu by thermodynamic compensation. Science. 2001;294:165–168. [PubMed: 11588263]
- 36.
- Zvereva MI, Ivanov PV, Teraoka Y. et al. Complex of tmRNA and EF-Tu: Unexpected modes of interaction. J Biol Chem. 2001;276:47702–47708. [PubMed: 11595738]
- 37.
- Karzai AW, Susskind MM, Sauer RT. SmpB, a unique RNA-binding protein essential for the peptide-tagging activity of SsrA (tmRNA). EMBO J. 1999;18:3793–3799. [PMC free article: PMC1171456] [PubMed: 10393194]
- 38.
- Wower IK, Zwieb CW, Guven S. et al. Binding and cross-linking of tmRNA to ribosomal protein S1, on and off the Escherichia coli ribosome. EMBO J. 2000;19:6612–21. [PMC free article: PMC305868] [PubMed: 11101533]
- 39.
- Sengupta J, Agrawal RK, Frank J. Visualization of protein S1 within the 30S ribosomal subunit and its interaction with messenger RNA. Proc Natl Acad Sci USA. 2001;98:11991–11996. [PMC free article: PMC59823] [PubMed: 11593008]
- 40.
- Sorokin A, Serror P, Pujic P, Azevedo V. et al. The Bacillus subtilis chromosome region encoding homologues of the Escherichia coli mssA and rpsA gene products. Microbiology. 1995;141:311319. [PubMed: 7704259]
- 41.
- Karzai AW, Sauer RT. Protein factors associated with the SsrA-SmpB tagging and ribosome rescue complex. Proc Natl Acad Sci USA. 2001;98:3040–3044. [PMC free article: PMC30603] [PubMed: 11248028]
- 42.
- Ando H, Kitabatake M, Inokuchi H. 10Sa RNA complements the temperature-sensitive phenotype caused by a mutation in the phosphoribosyl pyrophosphate synthetase (prs) gene in Escherichia coli. Genes Genet Syst. 1996;71:47–50. [PubMed: 8925474]
- 43.
- Nakano H, Goto S, Nakayashiki T. et al. Temperature-sensitive mutations in various genes of Escherichia coli K12 can be suppressed by the ssrA gene for 10Sa RNA (tmRNA). Mol Genet Genomics. 2001;265:615–621. [PubMed: 11459181]
- 44.
- Gillet R, Felden B. Transfer RNAAla recognizes transfer-messenger RNA with specificity; a functional complex prior to entering the ribosome? EMBO J. 2001;20:2966–2976. [PMC free article: PMC125487] [PubMed: 11387229]
- 45.
- Retallack DM, Friedman DI. A role for a small stable RNA in modulating the activity of DNA-binding proteins. Cell. 1995;83:227–235. [PubMed: 7585940]
- 46.
- Oh BK, Apirion D. 10Sa RNA, a small stable RNA of Escherichia coli, is functional. Mol Gen Genet. 1991;229:52–56. [PubMed: 1716727]
- 47.
- Muto A, Fujihara A, Ito KI. et al. Requirement of transfer-messenger RNA for the growth of Bacillus subtilis under stresses. Genes Cells. 2000;5:627–635. [PubMed: 10947848]
- 48.
- Huang C, Wolfgang MC, Withey J. et al. Charged tmRNA but not tmRNA-mediated proteolysis is essential for Neisseria gonorrhoeae viability. EMBO J. 2000;19:1098–1107. [PMC free article: PMC305648] [PubMed: 10698950]
- 49.
- Kirby JE, Trempy JE, Gottesman S. Excision of a P4-like cryptic prophage leads to Alp protease expression in Escherichia coli. J Bacteriol. 1994;176:2068–2081. [PMC free article: PMC205313] [PubMed: 7511583]
- 50.
- Karaolis DK, Johnson JA, Bailey CC. et al. A Vibrio cholerae pathogenicity island associated with epidemic and pandemic strains. Proc Natl Acad Sci USA. 1998;95:3134–3139. [PMC free article: PMC19707] [PubMed: 9501228]
- 51.
- Rettalack DM, Johnson LL, Friedman DI. Role for 10Sa RNA in the growth of lambda P22 hybrid phage. J Bacteriol. 1994;176:2082–2089. [PMC free article: PMC205314] [PubMed: 8144474]
- 52.
- Whitey J, Friedman D. Analysis of the role of trans-translation in the requirement of tmRNA for λimmP22 growth in Escherichia coli. J Bacteriol. 1999;181:2148–2157. [PMC free article: PMC93628] [PubMed: 10094693]
- 53.
- Ranquet C, Geiselmann J, Toussaint A. The tRNA function of SsrA contributes to controlling repression of bacteriophage Mu prophage. Proc Natl Acad Sci USA. 2001;98:10220–10225. [PMC free article: PMC56942] [PubMed: 11517307]
- 54.
- Jeffares DC, Poole AM, Penny D. Relics from the RNA World. J Mol Evol. 1998;46:18–36. [PubMed: 9419222]
- 55.
- Giegé R, Frugier M, Rudinger J. tRNA mimics. Curr Opin Struct Biol. 1998;8:286–293. [PubMed: 9666323]
- 56.
- Weiner AM, Maizels N. tRNA-like structures tag the 3' ends of genomic RNA molecules for replication: implications for the origin of protein synthesis. Proc Natl Acad Sci USA. 1987;84:7383–7387. [PMC free article: PMC299300] [PubMed: 3478699]
- 57.
- Park SJ, Hou YM, Schimmel P. A single base pair affects binding and catalytic parameters in the molecular recognition of a transfer RNA. Biochemistry. 1989;28:2740–2746. [PubMed: 2659081]
- 58.
- Westhof E, Dumas P, Moras D. Crystallographic refinement of yeast aspartic acid transfer RNA. J Mol Biol. 1985;184:119–145. [PubMed: 3897553]
- Summary
- Introduction
- Transcription and Maturation of tmRNA
- tmRNA Phylogeny
- The tRNA-Like Structure of tmRNA
- Aminoacylation of tmRNA
- Recruitment of Alanyl-tmRNA by the 70S Ribosome
- Additional Ligands of tmRNA
- Importance of ssrA and smpB for Survival and Pathogenesis
- tmRNA and Bacteriophages
- Molecular Origins of tmRNA Sequences
- Concluding Remarks
- Acknowledgments
- References
- Protein Tagging and Ribosome Rescue in Bacteria Requires the Recognition of Tran...Protein Tagging and Ribosome Rescue in Bacteria Requires the Recognition of Transfer-Messenger RNA by an Aminoacyl-tRNA Synthetase - Madame Curie Bioscience Database
- The Pathophysiological Role of Impaired Calcium Handling in Muscular Dystrophy -...The Pathophysiological Role of Impaired Calcium Handling in Muscular Dystrophy - Madame Curie Bioscience Database
- ER Calcium and ER Chaperones: New Players in Apoptosis? - Madame Curie Bioscienc...ER Calcium and ER Chaperones: New Players in Apoptosis? - Madame Curie Bioscience Database
- IL-10 Gene Polymorphisms in Transplantation - Madame Curie Bioscience DatabaseIL-10 Gene Polymorphisms in Transplantation - Madame Curie Bioscience Database
- MDM2: RING Finger Protein and Regulator of p53 - Madame Curie Bioscience Databas...MDM2: RING Finger Protein and Regulator of p53 - Madame Curie Bioscience Database
Your browsing activity is empty.
Activity recording is turned off.
See more...