NCBI Bookshelf. A service of the National Library of Medicine, National Institutes of Health.
Liedtke WB, Heller S, editors. TRP Ion Channel Function in Sensory Transduction and Cellular Signaling Cascades. Boca Raton (FL): CRC Press/Taylor & Francis; 2007.
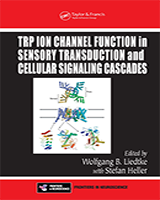
TRP Ion Channel Function in Sensory Transduction and Cellular Signaling Cascades.
Show detailsAbstract
In this chapter we address the puzzle of thermoTRP channel temperature and voltage sensitivity from a biophysical point of view. We deal with the problem through a classic thermodynamic approach, followed by a comparison of thermoTRPs’ behavior with our present knowledge regarding protein structure folding. On the basis of their temperature dependence and voltage dependence, we propose a general allosteric model for thermoTRP channel gating.
INTRODUCTION
Heat Receptors
TRP ion channels are one of the largest groups of ion channels, and this group is composed of six protein families [1–5]. With few exceptions, TRP channel subunits are predicted to have six transmembrane domains, and a subset of these channels, the thermoTRPs, is activated by distinct physiological temperatures. ThermoTRP channels have a tetrameric structure in which the subunits preferentially assemble into homomeric complexes [6,7]. Six members of the mammalian TRP ion channels respond to varied temperature thresholds. TRPV1, TRPV2, TRPV3, and TRPV4 are heat activated, whereas TRPM8 and TRPA1 are activated by cold [8–10]. The first member of this family to be cloned was the vanilloid receptor 1 (TRPV1) [11], which is mainly expressed in small-diameter sensory neurons of the trigeminal (TG) and dorsal root ganglia (DRG). Capsaicin (the active component of “hot” chili peppers), pH, and noxious thermal stimuli (~43°C) activate TRPV1 [12]. TRPV1 can be activated at room temperature when the pH is decreased to <6. This reduction in the temperature threshold for activation could be the mechanism involved in inflammatory pain because tissue acidification is induced in ischemia and inflammation [12]. More recently, Macpherson et al. [13] showed that allicin, a component of fresh garlic, is able to activate both TRPV1 and TRPA1 and is likely responsible for garlic’s pungency. Thus, TRPV1 can act as a transducer of noxious thermal and chemical stimuli in nociceptors, as has been confirmed in mice lacking this protein [14]. TRPV2, present in medium- to large-diameter DRG neurons and in motor neurons, is activated by high temperatures with a threshold of about 52°C [15–18]. TRPV3 is expressed in sensory neurons and keratinocytes [19–21]. It is activated by innocuous temperatures (~33°C), and its activity is further increased at noxious temperatures. TRPV3 is also markedly sensitized by repeated heat stimuli, but the mechanism accounting for this phenomenon is unknown; [9] the gradually sensitized initial phase is followed by an abrupt increase in current [22]. More recently, Moqrich et al. [23] found that the natural compound camphor specifically activates TRPV3 channels. TRPV4, previously known to be activated by osmotic pressure, was also shown to be activated by warm temperature stimuli (temperature threshold of ~35°C) and is expressed in DRGs, neurons, and keratinocytes, among other tissues [24–29].
Cold Receptors
TRPM8, which was cloned independently by two groups and characterized as a cold- and menthol-sensitive receptor, is expressed selectively in a small population of primary sensory neurons [30,31]. It is activated directly by gentle cooling (threshold ~22–30°C) and depolarizes sensory neurons [10,32]. TRPM8 is activated by menthol, the super-cooling agent icilin, and eucalyptol, albeit with lower efficacy and potency than menthol [30]. Strong cooling activates (<17°C) TRPA1 channels and has been suggested to be important in the transduction of strong (painful) cooling stimuli [33]. Isothiocyanate compounds, the active components of wasabi and mustard, activate TRPA1 channels, identifying this channel as the molecular target for the pungent action of mustard oils [34,35]. However, the involvement of this channel in cold sensitivity of sensory neurons has been questioned [36,34]. Bandell et al. [35] reported that noxious cold activates TRPA1 channels both in heterologous system and DRG cultures.
Thus, thermoTRPs provide us with the ability to evaluate temperature precisely over a wide range of temperatures, including those considered noxious. Their behavior suggests that either they are direct temperature sensors or they are activated by some ubiquitous temperature-sensitive molecular component extrinsic to the channel-forming protein. It is of importance therefore to unveil the molecular mechanisms that make these proteins so exquisitely temperature sensitive.
THERMOTRP BIOPHYSICS
Temperature Dependence
Channel gating is known to be affected by temperature. Q10 value is used to estimate the temperature dependence of a given system, taking the activity of the system at two different temperatures separated by 10°C. In the case of thermoTRPs, the Q10 could be easily obtained from the macroscopic currents using the following definition:
where I1 and I2 are the obtained currents at given temperatures T1 and T2. Although the Q10 obtained by this procedure is very useful as a comparative value, it lacks thermodynamic meaning.
A relationship between Q10 and the Arrehenius activation energy (Ea) can be obtained by measuring rate constants, k, at different temperatures since:
where A is a constant called the frequency factor. In the case of a first-order reaction k = 1/ where τ is the time constant it is easy to show that
where τ1 and τ2 are the time constants measured at T1 and T2, respectively, that can be written as:
ThermoTRP channels present exceptionally high Q10s (higher than 20). With a Q10 of ~26 for TRPV1 [37–39] and ~24 for TRPM8 [40], they far surpass the temperature dependence of the gating processes of other types of ion channels. With some notable exceptions, most channels’ gating processes have Q10 values of about 3 [41]. For example, in the Shaker K+ channel the inactivation process is highly temperature dependent. The inactivation rate increases with increasing temperature with a Q10 of 7 [42], a result that has been interpreted in terms of a temperature-induced stabilization of some structure(s) of the inactivation gate able to bind much more strongly to the channel than the other structures that the peptide can adopt [43]. Interestingly, when the inactivating peptide is used to block the channel, with a Q10 of 5, the peptide-association rate constant is much more temperature sensitive than the dissociation rate constant (Q10 ~1). The slow gating of the ClC0 channel shows a very strong temperature dependence (Q10 ~40), and this result has been interpreted in terms of a coupling of the slow gate with channel subunit interaction [44]. In general, a high Q10 for channel gating is compatible with large rearrangements of the protein induced by temperature. We notice here that in the case of TRPM8, the increase in ionic current is mediated by a decrease in temperature so the Q10, as defined in equation (1), would be less than one. This could be wrongly interpreted as a gating process having low temperature sensitivity. To avoid this, the Q10 for channels that respond to cold stimuli has to be defined as the reciprocal of the term on the right in equation (1). This is simply because equation (1) is not general. As we will discuss below, when rates are used to calculate Q10s [e.g., eq. (4)] their values are always higher than one.
For thermoTRPs such as TRPV1 and TRPM8, thermodynamic parameters such as the overall changes in enthalpy (H) and entropy (S) associated with channel opening reaction have been obtained considering a two-state model,
where C and O denote the closed and the open state and the voltage- and temperature-dependent α(T,V) and β(T,V) are the voltage- and temperature-dependent forward (activation) and backward (deactivation) rate constants, respectively. In this type of model, the thermodynamic meaning of the shift of the open probability (Po) vs. voltage curve with temperature is easy to visualize since
where
in this equation ΔG corresponds to the free energy of the closed-open reaction, given by the relation [45],
where z is the overall apparent gating charge, F is Faraday’s constant, and V the applied voltage. The enthalpy and entropy changes associated with the transition between closed and open states have been calculated using the van’t Hoff equation
and plotting lnK as a function of 1/T, assuming in this case that the term zFV/RT is negligible.
Some recent studies have addressed biophysics and thermodynamics of TRPV1, one of the molecular transducers of heat sensation and of its counterpart TRPM8, a cold receptor. Large values for transitional entropy, enthalpy, and Q10 were found in both channels (Table 21.1) [37,40], indicating large rearrangements in the channel structure during activation. A remarkable feature of these channels is that their openings are accompanied by very large enthalpic and entropic changes with the net result that the free energy is low [37,40]. Thus, the highly temperature-dependent close-open process takes place only because the large enthalpic change, which imparts the channel high-temperature sensitivity, is compensated by a large change in entropy so that the net free energy remains small. However, an increase in temperature induces an increase in ΔG for TRPM8 and a decrease in ΔG for TRPV1. This behavior is a consequence of the opposite signs of the entropic change, which is negative for TRPM8 and positive for TRPV1, indicating that for the cold receptor the closed state has greater entropy than the open state, whereas the opposite is true for TRPV1. Notice that for both TRPV1 and TRPM8 (Table 21.1), ΔH >> zFV because if z = 0.6 and V = 100 mV the term zFV is only 5.8 kcal/mol.
TABLE 21.1
Thermodynamic Parameters
Voltage Dependence
TRPV1 and TRPM8 are weakly voltage dependent, and the apparent number of gating charges (z) calculated from a Boltzmann fit (eq. 9) of the probability of opening (Po) vs. voltage data is 0.6–0.8 [40,46,47].
Where Pomax is the maximum probability of opening, z the apparent number of gating charges, and V0.5 is the potential at which Po = 0.5. TRPV3 and TRPV4 are also voltage dependent with zs in the range of those found for TRPV1 or TRPM8 [20,47,48]. The voltage dependence in these channels appears to be intrinsic to the channel-forming protein. The voltage sensor of these channels is, however, unknown, and a closer inspection of the predicted S4 segment of these channels reveals the presence of only one basic residue in TRPV1, TRPV3, and TRPV4 and three in TRPM8. It is possible that the weak voltage dependence showed by thermoTRPs is due to the scarcity of positive charges in the S4 domain. In the case of TRPV1 and TRPM8, temperature produces large (>100 mV) leftward shifts of the voltage activation curve upon heating and cooling, respectively [40,46].
We notice that when Po = 1/2, equations (5) and (6) imply that K = 1 and ΔG = 0. Inserting this condition in equation (7) gives that the voltage at which the probability is 1/2 is
where ΔH and ΔS can be obtained by plotting lnK vs. 1/ T using eq. (8) or V1/2 vs. T using eq. (10). Correa et al. [49] realized that the sign from the entropic change during channel opening can be obtained simply by measuring V1/2 at two different temperatures because from equation (10) we have
Voets et al. [46] have proposed that the temperature-dependent activities of TRPV1 and TRPM8 can be explained, at least in part, by the effects of temperature on the voltage-dependent gating. Because z in thermoTRPs is small, temperature changes promote large shifts of the voltage-activation curves when compared with a channel that has a strong voltage dependence like, for example, Shaker (Figure 21.1A to C). This observation appears to be valid even for more complicated kinetic schemes (Figure 21.1D and E). What makes thermoTRPs special is not their small z, but rather the enormous enthalpic change that imparts to these channels the capability to be gated by temperature as we discuss below. (Note also that Q10 directly relates to the transitional enthalpy, Ea through [eq.] 4.) We further argue that, given the ΔH and ΔS involved in the thermoTRP channels close-open reaction, the most efficient temperature-activated channel would be a voltage-independent one (Figure 21.1C), because temperature would be able to open (or close) the channel at all voltages.
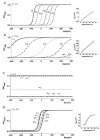
FIGURE 21.1
Open probabilities as a function of voltage at different temperatures. In a two-state model with ΔH = −86 kcal/mol, ΔS = −297cal/mol°K with (A) z = 2.5 and (B) z = 0.6 and (C) z = 0. For the eight-state allosteric (more...)
From eq. (5), (6), and (7) we have that the change in Po with temperature, assuming that ΔH and ΔS do not change with temperature, is given by the relation:
This function is zero when ΔG → ∝ and tends to (H-zFV)/(4RT [2]) when ΔG → 0. As thermoTRP channels exhibit relatively small ΔG values during the close-open transition, from equation (12) we see that to ensure large changes in Po with temperature, the only value that has to be large is ΔH. This, in turn, implies that the entropy term has to be large in order to make ΔG small in eq. (7).
PROTEIN DENATURATION, LIPIDS, AND THERMOTRP CHANNELS
The most plausible explanation for the effect of temperature on thermoTRP channels considers the existence of a temperature-sensing domain, a “temperature sensor” that would suffer large structural rearrangements upon temperature changes. Protein denaturation is also a highly temperature-dependent process, characterized by large entropic and enthalpic changes, whereas ΔG is relatively small (e.g., Privalov, 1989) [50]. This is another case in which a highly temperature-dependent process takes place only because the large enthalpic change is compensated by a large entropic change. Heat denaturation is the result of thermal excitation of the numerous disordered conformations accessible to the unfolded polypeptide chain. On the other hand, though the molecular details of cold denaturation remains unclear, it is usually attributed to a weakening of the hydrophobic effect caused by the temperature-dependent structure of bulk water. There are well-described examples of cold denaturation in the same range in which TRPM8 channels are being activated (26–10°C). Phosphoglicerate kinase is denaturated by cold at temperatures ≤30°C [51], and ApoAI denatures at temperatures lower than 25°C [52]. In these examples “denaturation” involves loss of tertiary and quaternary interactions, because the subunit interactions are the major target of the process. Among various intermolecular interactions, hydrophobic interactions are strongly temperature dependent as they decrease with temperature and even change signs at sufficiently low temperature [53]. As hydrophobic interactions are entropically driven, the negative ΔS obtained for TRPM8 during the activation process suggests that there is a net loss of hydrophobic interactions in the closed-to-open transition by temperature. It is important to consider that a protein that shows observable cold denaturation must be characterized by a substantial solvent exposure of apolar groups upon unfolding [54]. This suggests that it is possible that in thermoTRP channels, certain specialized regions of the channel suffer structural rearrangements driven by cold, whereas in TRPV1 channels, different rearrangements of this hypothetic “temperature sensor” are driven by heat.
Phase changes in the lipid bilayer membrane are well-known phenomena, and this phase transition can have implications in the gating of thermoTRPs induced by temperature. Experiments carried out on artificial lipid bilayer membranes indicate that ionic conductances mediated by the pore formers gramicidin and alamethicin do not show any peculiar effect at the transition temperature (tc) of the phospholipids [55,56]. On the other hand, the conductance induced by carriers such as valinomycin is essentially abolished when the temperature is reduced below the lipid phase transition temperature. These results suggest that carriers may freeze out into the membrane/water interface, and channels can be activated even below tc. Whether or not lipid phase changes play a role in thermoTRP channel gating is unknown, and it would be of interest to perform this type of study by incorporating thermoTRP channels into lipid bilayers composed of lipids with well defined tc. Regarding possible effects of membrane lipid composition on thermoTRP channel gating, Liu and colleagues showed that cholesterol depletion did not have a significant effect on heat response of the TRPV1 channel but that cholesterol enrichment shifted the half activation temperature in 4°C [37].
MULTISTATE NATURE OF THERMOTRPS’ OPENING PROCESS
At the single channel level for TRPV1, as temperature increases more openings occur, bursts are prolonged, and quiescent (gaps) periods between bursts are shortened [37]. Temperature activates the TRPV1 channel by increasing the burst duration (Q10 ~32) and shortening the gaps (Q10 = 7). Thus, most of the TRPV1 temperature dependence resides in the burst elongation, because the Q10 of the overall single channel activity (Po) was estimated to be 27. Analysis of the channel activity within a burst reveals at least three closed and three open states, the duration of which are only weakly temperature dependent. Capsaicin and pH produce a pattern of single-channel activity similar to that found when temperature is increased [57,58]. On the other hand, at the macroscopic current level, for both TRPM8 and TRPV1 the time-dependent changes in conductance due to a membrane depolarization or repolarization occurred with bi-exponential kinetics [59,40]. In the case of TRPV1, temperature affects preferentially the time constant of the slowest component of the current activation (Q10 = 9) [46]. Temperature only slightly modifies the time constant for the deactivation of TPRV1 channels (Q10 = 1.4). On the other hand, for TRPM8 the time constant of the slowest component of the current deactivation is steeply temperature dependent (Q10 = 4 – 9) [40,46], whereas the slowest component of current activation has only a Q10 of 1.4. The deactivation time constant in TRPM8 decreases as temperature decreases, indicating that closing rates are the parameters affected by temperature and explaining, at least in part, why cooling augments TRPM8-induced macroscopic currents, giving rise to an anomalous (<1) Q10. The Q10 for the overall channel gating is ~25 (see Table 21.1), indicating that the temperature-dependent components of the macroscopic channel kinetics have a lower temperature dependence than the overall activation of TRPV1 or TRPM8. The interpretation of the effect of temperature on the activation kinetics can be difficult if the activation pathway is multistep and ramified, which appears to be the case with thermoTRP channels (see below and Ryu et al.; Brauchi et al.) [40,58]. In that case, Schoppa and Sigworth [60] demonstrated that only for large depolarizations where the backward rate constants are negligible, the time constant calculated from the macroscopic current is a measure of the reciprocal value of the slowest rate constant in the activation path. In the case of TRPV1, the lower Q10 (~9) of the activation time constant (τa) [46] compared to that obtained for the mean burst time (~32) may reflect the fact that τa is the inverse of a composite of rate constants with widely different values and temperature sensitivities.
A MINIMUM MODEL THAT EXPLAINS THE CHANGE IN LENGTH OF BURSTS AND GAPS WITH TEMPERATURE
The simplest kinetic scheme able to explain single-channel current records in which the opening occurs in bursts separated by gaps is a model containing one open and two closed states:
In this model, the bursts consist in a sojourn between the Open and Closed2 states and the gaps in a sojourn in the Closed1 state. To ensure this, the constants k+ and k− have to be much larger than α and β. To predict heat-induced changes in the length of bursts and gaps, α and β have to be highly temperature dependent.
According to Eyring’s transition state theory, the kinetic constants are related to the enthalpy and entropy of activation by the following equations
where ΔH† and ΔS† are the enthalpy and entropy of activation of each rate constant, respectively, and is the prefactor rate that depends on the type of process and here will be assumed to be 106 s−1 [61]. If the reaction between Closed2 and Open is in equilibrium with respect to Closed1–Closed2 then termination of the burst will be determined by the rate constant β and k1/k−1 and the termination of a gap by α. Introducing the appropriate values (k+ = k− =104 s−1, ΔHα† = 39.5 kcal/mol, ΔSα† = 100 cal/mol°K, ΔHβ† = −70 kcal/mol, and ΔSα† = −243 cal/mol°K) in this model, the behavior of the single-channel currents of the TRPV1 channel measured by Liu et al. [37] can be reproduced (Figure 21.2A).
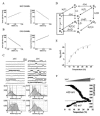
FIGURE 21.2
(A) Relationship between the mean time of gaps and bursts with temperature in the model on scheme I. The values used were k+ = k-= 104 s−1, ΔHα† = 39.5 kcal/mol, ΔSα†= 100 cal/mol°K, ΔH (more...)
If the values of ΔH† and ΔS† are exchanged between α and β, we found that the temperature dependence of the gaps is higher than that of the bursts (Figure 21.2B) and it is possible to reproduce qualitatively TRPM8 single channel behavior when the temperature is changed (Figure 21.2C).
AN ALLOSTERIC MODEL FOR THERMOTRP ACTIVATION
In TRPM8 channels, we found that a simple way to explain our data was to use an allosteric activation mechanism for both voltage and temperature dependence [40]. In this model, we assumed the existence of a “temperature sensor” that has, as does the voltage sensor, resting and activated states (Figure 21.2D). We propose that there is an allosteric linkage between the voltage and temperature sensors or, in other words, neither voltage nor temperature is strictly necessary for channel activation (Figure 21.2D, boxed section). This model can reproduce the steady-state behavior of TRPM8 over a wide range of conditions, with the assumption that activation of voltage sensors and temperature sensors additively affect the energy of the closed-open transitions. The allosteric model successfully accounts for the shift in V0.5 with temperature, noticing that the value of V0.5 tends to saturate at high temperatures and low temperatures (cf. eq. [10]; Figure 21.1D, E, and 21.2F; Voets et al; Brauchi et al.) [40,46]. This type of model has been very successful in explaining the behavior of another channel activated by two stimuli: the Ca2+- and voltage-activated potassium channel (Slo1; reviewed in Magleby) [62].
Testing the Allosteric Model
Figure 21.2C shows the presence of multiple open and closed states in the TRPM8 channel. How these states are connected is not known and requires detailed study of the single channel gating kinetics, in particular the possible correlation between open and closed states [63]. The presence of several open and closed states gives, however, support to the model shown in Figure 21.2D. The allosteric model predicts that in the absence of stimuli (i.e., at negative voltages and high temperatures), the channel should be confined to the equilibrium between states C0 and O0 determined by the equilibrium constant L (i.e., the channel can open in the absence of activated voltage or temperature sensors). In this case the probability of opening is given by the equation:
According to the allosteric model, at sufficiently negative voltages voltage sensors should remain in the resting state even when temperature is decreased. Figure 21.2F shows that at negative voltages (−60 mV), TRPM8-induced current is highly temperature sensitive albeit with a lower temperature threshold when compared to the data obtained at +60 mV. Thus, as the allosteric model predicts, a decrease in temperature can activate TRPM8 channels when all voltage sensors are in the resting state.
Because the channel is a homotetramer, it is likely to contain four voltage sensors and the same number of temperature-sensing structures, and this clearly will increase the number of states to at least 50. The situation, however, is not desperate, because the number of parameters can be reduced (and determined) working under extreme voltages or temperatures and measuring and characterizing single-channel and macroscopic currents. It is necessary to understand thermoTRPs’ gating mechanisms in terms of models that include the large number of open and closed states (e.g., Liu et al.) [37]. Allosteric models offer a natural explanation to the single-channel data, assuming that the channel has multiple heat-sensitive sites distributed over the subunits. Activation of one site brings about a short burst of activity. As temperature is increased, more sites become active, and if they interact allosterically, this interaction will produce elongation of the bursts as observed experimentally in TRPV1 [37]. Allosteric models have been successful in explaining the workings of other channels gated by several stimuli (e. g., Horrigan and Aldrich; Orio and Latorre) [64,65] and can be particularly useful to describe the complex regulation of TRPM8 by agonists such as PIP2 and cooling agents and that of TRPV1 by capsaicin and pH.
REFERENCES
- 1.
- Clapham DE. TRP channels as cellular sensors. Nature. 2003;426:517. [PubMed: 14654832]
- 2.
- Montell C. An end insight to a long TRP. Neuron. 2001;30(1):3. [PubMed: 11343636]
- 3.
- Montell C, Birnbaumer L, Flockerzi V. The TRP channels, a remarkably functional family. Cell. 2002;108:595. [PubMed: 11893331]
- 4.
- Moran MM, Xu H, Clapham DE. TRP ion channels in the nervous system. Curr Opin Neurobiol. 2004;14(3):362. [PubMed: 15194117]
- 5.
- Voets T, Talavera K, Owsianik G, Nilius B. Sensing with TRP channels. Nature Chem Biol. 2005;1(2):85. [PubMed: 16408004]
- 6.
- Kedei N, et al. Analysis of quaternary structure of vanilloid receptor 1. J Biol Chem. 2001;276(30):28613. [PubMed: 11358970]
- 7.
- Hellwig N, et al. Homo- and heteromeric assembly of TRPV channel subunits. J Cell Sci. 2005;118(5):917. [PubMed: 15713749]
- 8.
- Tominaga M, Caterina MJ. Thermosensation and pain. J Neurobiol. 2004;61(1):3. [PubMed: 15362149]
- 9.
- Benham CD, Gunthorpe MJ, Davis JB. TRPV channels as temperature sensors. Cell Calcium. 2003;33:479. [PubMed: 12765693]
- 10.
- McKemy DD. How cold is it? TRPM8 and TRPA1 in the molecular logic of cold sensation. Mol Pain. 2005;1(1):16. [PMC free article: PMC1087877] [PubMed: 15847696]
- 11.
- Caterina MJ, et al. The capsaicin receptor: a heat-activated ion channel in the pain pathway. Nature. 1997;389:816. [PubMed: 9349813]
- 12.
- Tominaga M, et al. The cloned capsaicin receptor integrates multiple pain-producing stimuli. Neuron. 1998;21:531. [PubMed: 9768840]
- 13.
- Macpherson LJ, et al. The pungency of garlic: activation of TRPA1 and TRPV1 in response to allicin. Curr Biol. 2005;15:929. [PubMed: 15916949]
- 14.
- Caterina MJ, et al. Impaired nociception and pain sensation in mice lacking the capsaicin receptor. Science. 2000;288:306. [PubMed: 10764638]
- 15.
- Caterina MJ, et al. A capsaicin-receptor homologue with a high threshold for noxious heat. Nature. 1999;398:436. [PubMed: 10201375]
- 16.
- Ma HT, et al. Assessment of the role of the inositol 1,4,5-trisphosphate receptor in the activation of transient receptor potential channels and store-operated Ca2+ entry channels. J Biol Chem. 2001;276(22):18888. [PubMed: 11259416]
- 17.
- Ahluwalia J, Rang H, Nagy I. The putative role of vanilloid receptor–like protein-1 in mediating high-threshold noxious heat-sensitivity in rat cultured primary sensory neurons. Eur J Neurosci. 2002;16(8):1483. [PubMed: 12405961]
- 18.
- Lewinter RD, et al. Immunoreactive TRPV-2 (VRL-1), a capsaicin receptor homolog, in the spinal cord of the rat. J Comp Neurol. 2004;470(4):400. [PubMed: 14961565]
- 19.
- Smith GD, et al. TRPV3 is a temperature-sensitive vanilloid receptor–like protein. Nature. 2002;418:186. [PubMed: 12077606]
- 20.
- Xu H, et al. TRPV3 is a calcium-permeable temperature-sensitive cation channel. Nature. 2002;418:181. [PubMed: 12077604]
- 21.
- Peier AM, et al. A heat-sensitive TRP channel expressed in keratinocytes. Science. 2002a;296(5575):2046. [PubMed: 12016205]
- 22.
- Chung MK, Guler AD, Caterina MJ. Biphasic currents evoked by chemical or thermal activation of the heat-gated ion channel, TRPV3. J Biol Chem. 2005;280(16):15928. [PubMed: 15722340]
- 23.
- Moqrich A, et al. Impaired thermosensation in mice lacking TRPV3, a heat and camphor sensor in the skin. Science. 2005;307(5714):1468. [PubMed: 15746429]
- 24.
- Liedtke W, et al. Vanilloid receptor–related osmotically activated channel (VR-OAC), a candidate vertebrate osmoreceptor. Cell. 2000;103(3):525. [PMC free article: PMC2211528] [PubMed: 11081638]
- 25.
- Strotmann R, et al. OTRPC4, a nonselective cation channel that confers sensitivity to extracellular osmolarity. Nat Cell Biol. 2000;2(10):695. [PubMed: 11025659]
- 26.
- Güler AD, et al. Heat-evoked activation of the ion channel, TRPV4. J Neurosci. 2002;22(15):6408. [PMC free article: PMC6758176] [PubMed: 12151520]
- 27.
- Watanabe H, et al. Heat-evoked activation of TRPV4 channels in a HEK293 cell expression system and in native mouse aorta endothelial cells. J Biol Chem. 2002;277(49):47044. [PubMed: 12354759]
- 28.
- Chung MK, et al. 2-aminoethoxydiphenyl borate activates and sensitizes the heat-gated ion channel TRPV3. J Neurosci. 2004a;24(22):5177. [PMC free article: PMC6729202] [PubMed: 15175387]
- 29.
- Chung MK, et al. TRPV3 and TRPV4 mediate warmth-evoked currents in primary mouse keratinocytes. J Biol Chem. 2004b;279(20):21569. [PubMed: 15004014]
- 30.
- McKemy DD, Neuhausser WM, Julius D. Identification of a cold receptor reveals a general role for TRP channels in thermosensation. Nature. 2002;416(6876):52. [PubMed: 11882888]
- 31.
- Peier AM, et al. A TRP channel that senses cold stimuli and menthol. Cell. 2002b;108(5):705. [PubMed: 11893340]
- 32.
- Reid G. ThermoTRP channels and cold sensing: what are they really up to. Pflügers Arch. 2005 June 17 [PubMed: 16075243]
- 33.
- Story GM, et al. ANTKTM1, a TRP-like channel expressed in nociceptive neurons, is activated by cold temperatures. Cell. 2003;112:819. [PubMed: 12654248]
- 34.
- Jordt SE, et al. Mustard oils and cannabinoids excite sensory nerve fibres through the TRP channel ANTKTM1. Nature. 2004;427:260. [PubMed: 14712238]
- 35.
- Bandell M, et al. Noxious cold ion channel TRPA1 is activated by pungent compounds and bradykinin. Neuron. 2004;41(6):849. [PubMed: 15046718]
- 36.
- Babes A, Zorzon D, Reid G. Two populations of cold-sensitive neurons in rat dorsal root ganglia and their modulation by nerve growth factor. Eur J Neurosci. 2004;20(9):2276. [PubMed: 15525269]
- 37.
- Liu B, Hui K, Qin F. Thermodynamics of heat activation of single capsaicin ion channels VR1. Biophys J. 2003;85:2988. [PMC free article: PMC1303577] [PubMed: 14581201]
- 38.
- Nagy I, Rang HP. Similarities and differences between the responses of rat sensory neurons to noxious heat and capsaicin. J Neurosci. 1999;19(24):10647. [PMC free article: PMC6784946] [PubMed: 10594048]
- 39.
- Vlachova V, et al. Functional role of C-terminal cytoplasmic tail of rat vanilloid receptor 1. J Neurosci. 2003;23(4):1340. [PMC free article: PMC6742269] [PubMed: 12598622]
- 40.
- Brauchi S, Orio P, Latorre R. Clues to understanding cold sensation: thermodynamics and electrophysiological analysis of the cold receptor TRPM8. Proc Natl Acad Sci USA. 2004;101(43):15494. [PMC free article: PMC523461] [PubMed: 15492228]
- 41.
- Hille B. Ionic channels of excitable membranes. Sinauer Associates; Sunderland, MA: 2001.
- 42.
- Nobile M, et al. Exp Brain Res. 1. Vol. 114. 1997. Fast inactivation of Shaker K+ channels is highly temperature dependent; p. 138. [PubMed: 9125459]
- 43.
- Murrell-Lagnado RD, Aldrich RW. Energetics of Shaker K channels block by inactivation peptides. J Gen Physiol. 1993;102(6):977. [PMC free article: PMC2229186] [PubMed: 8133246]
- 44.
- Pusch M, Ludewig U, Jentsch TJ. Temperature dependence of fast and slow gating relaxations of CIC-O chloride channels. J Gen Physiol. 1997;109(1):105. [PMC free article: PMC2217054] [PubMed: 8997669]
- 45.
- Lecar H, Ehrenstein G, Latorre R. Mechanism for channel gating in excitable bilayers. Ann NY Acad Sci. 1975;264:304. [PubMed: 1062959]
- 46.
- Voets T, et al. The principle of temperature-dependent gating in cold- and heat-sensitive TRP channels. Nature. 2004;430(7001):748. [PubMed: 15306801]
- 47.
- Nilius B, et al. Gating of TRP channels: a voltage connection. J Physiol. 2005;567(1):35. [PMC free article: PMC1474154] [PubMed: 15878939]
- 48.
- Chung MK, Guler AD, Caterina MJ. Biphasic currents evoked by chemical or thermal activation of the heat-gated ion channel, TRPV3. J Biol Chem. 2005;280(16):15928. [PubMed: 15722340]
- 49.
- Correa AM, Bezanilla F, Latorre R. Gating kinetics of batrachotoxin-modified Na+ channels in the squid giant axon. Voltage and temperature effects. Biophys J. 1992;61(5):1332. [PMC free article: PMC1260396] [PubMed: 1318096]
- 50.
- Privalov PL. Thermodynamic problems of protein structure. Annu Rev Biophys Biophys Chem. 1989;18:47. [PubMed: 2660833]
- 51.
- Griko YV, Venyaminov SY, Privalov PL. Heat and cold denaturation of phosphoglycerate kinase (interaction of domains). FEBS Lett. 1989;244(2):276. [PubMed: 2646151]
- 52.
- Gursky O, Atkinson D. Thermal unfolding of human high-density apolipoprotein A-1: implications for a lipid-free molten globular state. Proc Natl Acad Sci USA. 1996;93(7):2991. [PMC free article: PMC39748] [PubMed: 8610156]
- 53.
- Privalov PL, Gill SJ. Stability of protein structure and hydrophobic interaction. Adv Protein Chem. 1988;39:191. [PubMed: 3072868]
- 54.
- Privalov PL. Cold denaturation of proteins. Crit Rev Biochem Mol Biol. 1990;25(4):281. [PubMed: 2225910]
- 55.
- Krasne S, Eisenman G, Szabo G. Freezing and melting of lipid bilayers and the mode of action of nonactin, valinomycin, and gramicidin. Science. 1971;174(7):412. [PubMed: 5111995]
- 56.
- Boheim G, Hanke W, Eibl H. Lipid phase transition in planar bilayer membrane and its effect on carrier- and pore-mediated ion transport. PNAS. 1980;77(6):3403. [PMC free article: PMC349624] [PubMed: 6158046]
- 57.
- Hui K, Liu B, Qin F. Capsaicin activation of the pain receptor, VR1: multiple open states from both partial and full binding. Biophys J. 2003;84:2957. [PMC free article: PMC1302858] [PubMed: 12719227]
- 58.
- Ryu S, Liu B, Qin F. Low pH potentiates both capsaicin binding and channel gating of VR1 receptors. J Gen Physiol. 2003;122:45. [PMC free article: PMC2234467] [PubMed: 12835470]
- 59.
- Ahern GP, Premkumar LS. Voltage-dependent priming of rat vanilloid receptor: effects of agonist and protein kinase C activation. J Physiol. 2002;545(2):441. [PMC free article: PMC2290684] [PubMed: 12456824]
- 60.
- Schoppa NE, Sigworth FJ. Activation of Shaker potassium channels. III. An activation gating model for wild-type and V2 mutant channels. J Gen Physiol. 1998;111(2):313. [PMC free article: PMC2222769] [PubMed: 9450946]
- 61.
- Yang WY, Gruebele M. Folding at the speed limit. Nature. 2003;423(6936):193. [PubMed: 12736690]
- 62.
- Magleby KL. Gating mechanism of BK (Slo1) channels: so near, yet so far. J Gen Physiol. 2003;121(2):81. [PMC free article: PMC2217328] [PubMed: 12566537]
- 63.
- McManus OB, Blatz AL, Magleby KL. Inverse relationship of the durations of adjacent open and shut intervals for C1 and K channels. Nature. 1985;317(6038):625. [PubMed: 2414664]
- 64.
- Horrigan FT, Aldrich RW. Coupling between voltage sensor activation, Ca2+ binding and channel opening in large conductance (BK) potassium channels. J Gen Physiol. 2002;120(3):267. [PMC free article: PMC2229516] [PubMed: 12198087]
- 65.
- Orio P, Latorre R. Differential effects of beta 1 and beta 2 subunits on BK channel activity. J Gen Physiol. 2005;125(4):395. [PMC free article: PMC2217511] [PubMed: 15767297]
- 66.
- Horn R, Vandenberg CA, Lange K. Statistical analysis of single sodium channels. Effects of N-bromoacetamide. Biophys J. 1984;45(1):323. [PMC free article: PMC1435274] [PubMed: 6324912]
- 67.
- Colquhoun D, Hawkes AG. On the stochastic properties of single ion channels. Proc R Soc Lond. 1981;211:205. [PubMed: 6111797]
- Review ThermoTRP channels as modular proteins with allosteric gating.[Cell Calcium. 2007]Review ThermoTRP channels as modular proteins with allosteric gating.Latorre R, Brauchi S, Orta G, Zaelzer C, Vargas G. Cell Calcium. 2007 Oct-Nov; 42(4-5):427-38. Epub 2007 May 17.
- Review Thermodynamic and structural basis of temperature-dependent gating in TRP channels.[Biochem Soc Trans. 2021]Review Thermodynamic and structural basis of temperature-dependent gating in TRP channels.Diaz-Franulic I, Verdugo C, Gonzalez F, Gonzalez-Nilo F, Latorre R. Biochem Soc Trans. 2021 Nov 1; 49(5):2211-2219.
- Review Progress in the Structural Basis of thermoTRP Channel Polymodal Gating.[Int J Mol Sci. 2023]Review Progress in the Structural Basis of thermoTRP Channel Polymodal Gating.Fernández-Ballester G, Fernández-Carvajal A, Ferrer-Montiel A. Int J Mol Sci. 2023 Jan 1; 24(1). Epub 2023 Jan 1.
- Review Quantifying and modeling the temperature-dependent gating of TRP channels.[Rev Physiol Biochem Pharmacol....]Review Quantifying and modeling the temperature-dependent gating of TRP channels.Voets T. Rev Physiol Biochem Pharmacol. 2012; 162:91-119.
- Review Structure of thermally activated TRP channels.[Curr Top Membr. 2014]Review Structure of thermally activated TRP channels.Cohen MR, Moiseenkova-Bell VY. Curr Top Membr. 2014; 74:181-211.
- Voltage and Temperature Gating of ThermoTRP Channels - TRP Ion Channel Function ...Voltage and Temperature Gating of ThermoTRP Channels - TRP Ion Channel Function in Sensory Transduction and Cellular Signaling Cascades
Your browsing activity is empty.
Activity recording is turned off.
See more...