NCBI Bookshelf. A service of the National Library of Medicine, National Institutes of Health.
Liedtke WB, Heller S, editors. TRP Ion Channel Function in Sensory Transduction and Cellular Signaling Cascades. Boca Raton (FL): CRC Press/Taylor & Francis; 2007.
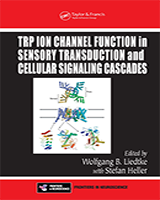
TRP Ion Channel Function in Sensory Transduction and Cellular Signaling Cascades.
Show detailsINTRODUCTION
Membrane ion channels are critical mediators of vascular endothelial and smooth muscle cell function and regulate diverse properties of blood vessels such as arterial tone, angiogenesis, and permeabilty. Although physiological roles for many ion channels and transporters are well established, the functional significance of transient receptor potential (TRP) channels in the vasculature is just beginning to be elucidated. This chapter summarizes the current understanding of these cation channels in vascular function. For a more in-depth overview of TRP channel biology, the reader is directed to several excellent general reviews covering this topic [1–4].
As shown in Table 26.1, the mammalian TRP superfamily of cation channels contains at least 22 genes grouped into three major subfamilies based on sequence homology: TRPV (vanilloid), TRPC (canonical), and TRPM (melastatin). Three additional subfamilies (the “distant TRPs”), TRPP (polycystin), TRPML (mucolipin), and TRPA have been proposed, bringing the total number of TRP-related proteins to around 30. Although these channels were initially described in sensory neurons, it is now thought that most cell types express several TRP genes. TRP proteins are expressed as six transmembrane-domain polypeptide subunits, and it is believed that four subunits assemble in the plasma membrane to form functional channels. All TRP channels are cation permeable, and most are not selective for monovalent versus divalent ions. Exceptions include TRPV5 and TRPV6, which display significant specificity for Ca2+ ions, and TRPM4 and TRPM5, which are highly selective for monovalent cations and impermeant to Ca2+ TRP channels are activated by a variety of stimuli, including changes in pressure, temperature, osmolarity, and intracellular Ca2+ Fatty acids and receptor-dependent vasoconstrictor agonists also activate vascular TRP channels. This diversity of ionic conductivity and activating mechanisms is consistent with the possibility that members of the TRP superfamily may contribute to regulation of a variety of physiological systems.
TABLE 26.1
Summary of TRP Channels in Vascular Tissue
Elucidation of functional roles for TRP channels in vascular cells may be hindered by the complex molecular biology of the superfamily. Biophysical properties of TRP channels have been investigated in patch-clamp experiments employing cultured cells expressing cloned TRP subunit genes. Under these conditions, most functional channels assemble from four identical TRP subunits. However, when multiple TRP subunits are coexpressed, assembly of tetramers composed of two or more TRP subunit proteins can form heteromeric channels [5,6] with novel properties [7,8]. Because most cells express multiple TRP subunits, it is likely that heteromeric channels exist in vivo. Furthermore, splice variants of TRP mRNAs in smooth muscle have been reported [9], potentially increasing the number of individual subunits available for coassembly. Because TRP molecular variety could result in an assortment of channels with a wide array of functional properties in native cells, unraveling the physiological consequences of TRP channel diversity presents a major challenge. In the following paragraphs, evidence for the presence and possible functional roles of TRP channels in vascular smooth muscle and endothelial cells are discussed.
TRP CHANNEL FUNCTION IN VASCULAR SMOOTH MUSCLE CELLS
Vasoconstrictor Agonist-Induced TRP Channel Activation in Smooth Muscle
Vascular smooth muscle cells contract when exposed to a variety of excitatory neurotransmitters and hormones such as endothelin, histamine, norepinephrine, serotonin, and purine and pyrimidine nucleotides. Many of these constrictor agonists bind to G-protein- or tyrosine kinase–coupled receptors that activate phospholipase C (PLC) to initiate the conversion of phosphatidylinositol 4,5-bisphosphate into inositol-1,4,5-trisphosphate (IP3) and diacylglycerol (DAG) at the sarcolemmal membrane [10]. PLC-coupled agonists raise cytosolic Ca2+ ([Ca2+]c) with a characteristic early transient phase followed by a secondary sustained phase. IP3-mediated Ca2+ release from the sarcoplasmic reticulum contributes to the early large increase in [Ca2+]c [11,12] Ca2+ influx across the sarcolemmal membrane contributes to the maintenance of cytosolic Ca2+ during the sustained phase as evidenced by the fact that elevated [Ca2+]c and vasoconstriction are not maintained in the absence of extracellular Ca2+ [13] Extracellular Ca2+ influx occurs in part through well-defined voltage-gated Ca2+ channels [14] as well as through channels gated independently of the membrane potential [15]. These nonselective cation channels coupled to PLC, possibly through DAG, appear to play a role in the extracellular Ca2+ influx by directly permitting Ca2+ influx or by permitting the influx of monovalent cations that depolarize the smooth muscle cell [16–19]. The molecular identities of these channels are not yet known.
Accumulating evidence supports the idea that some of these channels may in fact be mammalian homologues of TRP channels first identified in photoreceptors of Drosophila melanogaster. In Drosophila, light causes a sustained depolarization of photoreceptors through a signal transduction pathway mediated by PLC [20]. Two conductances—one that is Ca2+ selective and another that is permeable to both Ca2+ and Na+—are responsible for light-induced depolarization. Gene products encoded by trp and trpl (TRP-like) are believed to be responsible for these conductances by forming membrane channels that have structural homologues with voltage-gated channels [21]. Of the three identified mammalian TRP channel families, members of the TRPC family share the greatest similarity to Drosophila TRP and TRPL channels [4]. Thus, TRPC channels may be responsible for voltage-independent extracellular Ca2+ influx in vascular smooth muscle exposed to PLC-coupled agonists.
There is evidence to show that most TRPC channels (TRPC2 is the exception) are activated by stimulation of PLC, formation of DAG, or exposure to DAG analogues. TRPC channels appear to be widespread in the mammalian vasculature and mRNA, for all seven TRPC channels have been detected in venous and arterial smooth muscle from a variety of vascular beds [3,9,22,23]. TRPC1, TRPC3, and TRPC6 are abundant in vascular smooth muscle, and these proteins have been detected by Western blot in every vascular bed so far examined. TRPC channels are divided into four subfamilies based on amino acid homology: TRPC1, TRPC2, TRPC4/5 (60 percent homology), and TRPC3/6/7 (~80 percent homology) [24]. Functional channels form as tetramers of individual TRPC proteins. All TRPC subunits can combine to form homomeric channels [25], and heteromeric channels can result when TRPC proteins combine with other members of their own subfamily [6] or when TRPC1 combines with either TRPC4/5 [6,8] or TRPC3 [26,27]. The combinatorial rules governing the assembly of TRPC proteins into functional channels in vascular smooth muscle cells are not known. It is likely that TRPC proteins assemble to form both homomeric and heteromeric channels.
Elucidation of TRPC channel function in native vascular cells has been impeded by the complex molecular biology of the TRP superfamily and by the lack of selective pharmacological inhibitors. A few studies using genetic methods to downregulate TRPC expression in intact vascular tissue have yielded valuable information. For example, Inoue et al. [28] observed that exposure to phenylephrine (PE, a vasoconstrictor agonist that activates α1-adrenoreceptors coupled to PLC) produced a current in vascular smooth muscle cells isolated from the rabbit portal vein that displayed dual rectification; the channel was permeable to divalent cations and had a unitary conductance of 25 to 30 pS. This channel could also be activated by DAG, and the channel activity was potentiated by flufenamte (a nonspecific cation channel inhibitor that “uniquely” enhances α1-adrenoreceptor nonselective cation currents in the rabbit portal vein) and by extracellular Ca2+. The channel was inhibited by other nonselective cation channel inhibitors including Cd2+, La3+, Gd3+, SK&F96365, and amiloride. PE-induced currents with virtually identical properties to those found in the native cells were recorded from HEK cells overexpressing cloned TRPC6. Downregulation of TRPC6 expression in primary cultured portal vein myocytes using antisense oligodeoxynucleotides resulted in suppression of α1-adrenoreceptor-activated cation currents. These findings show that TRPC6 channels are critical mediators of PE-induced responses in portal vein smooth muscle cells. A current with biophysical and pharmacological properties similar to that described by Inoue et al. [28] was recorded in the smooth muscle–derived A7r5 cell line exposed to vasopressin or membrane-permeable analogues of DAG [29]. Northern blots of cDNA probes for all seven TRPCs revealed that only TRPC1 and TRPC6 were present in these cells. Because the current showed properties characteristic of TRPC6, and because there is no evidence to support the presence of a TRPC1/6 heteromeric channel, it is likely that the agonist-induced current was mediated by TRPC6 channels.
Uridine trisphosphate (UTP) causes depolarization and contraction of both coronary and cerebrovascular smooth muscle cells [30,31]. We found that antisense oligonucleotide suppression of TRPC3 expression in cerebral arteries resulted in a diminished UTP-induced depolarization and vasoconstriction [31]. In addition, UTP-activated whole-cell cation currents were greatly suppressed in cerebral artery myocytes following antisense-mediated TRPC3 downregulation. Furthermore, although about 50 percent of the UTP-induced, TRPC3-mediated constriction appears to be due to the depolarization and activation of L-type calcium channels, our recent observations suggest that much of the balance of the vasoconstriction is due to direct calcium entry via the TRPC3 channel per se [32] (see Figure 26.1).
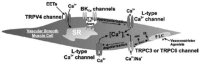
FIGURE 26.1
TRP channels that are receptor coupled and that directly or indirectly mediate calcium entry in vascular smooth muscle cells.
Findings from our laboratory also show that, in rat cerebral arteries, TRPC3 and TRPC6 are coupled to distinct excitatory stimuli. TRPC3 (and not TRPC6) is involved in UTP-mediated depolarization and vasoconstriction, whereas TRPC6 (and not TRPC3) is involved in pressure-induced depolarization and vasoconstriction [31,33]. These findings suggest that individual TRPC channel isoforms may be activated by different stimuli (i.e., agonist versus pressure) in the same vascular bed.
In addition to being involved in normal vascular smooth muscle cell function, a few studies have shown that TRPC expression is altered in vascular smooth muscle during pathological conditions or during environmental stress. For example, Yu et al. [22] showed that TRPC3 and TRPC6 expression was significantly greater in the pulmonary artery smooth muscle cells of patients with idiopathic pulmonary hypertension when compared with healthy individuals or patients with secondary pulmonary hypertension. Other investigators reported that arteries undergoing organ culture and balloon dilation showed increased TRPC1 and TRPC6 expression, whereas TRPC3 expression was decreased [34]. Another study demonstrated that chronic hypoxia increased TRPC1 and TRPC6 expression in intralobar artery smooth muscle cells in rabbits [35]. The implication of this observed plasticity in TRPC expression is that TRPC channels are involved in normal vascular smooth muscle function and may also play a role in vascular dysfunction and pathology. Future exploration of agonist-TRPC channel interaction will likely yield exciting new information on vascular smooth muscle cell function as well as insight into a variety of vascular pathologies.
Vasodilator Response to EETs Mediated by TRP Channel Activation in Smooth Muscle
TRPV4 is present in arterial cells. A recent report by Earley et al. [36] indicates that TRPV4 is involved in vasodilation induced by epoxyeicosatrienoic acid compounds (EETs). These vasodilatory factors are produced by the vascular endothelium and have been proposed to be one form of endothelium-derived hyperpolarizing factor. Earley et al. found that EET-induced vasodilation of cerebral arteries involves a cascade of responses beginning with the activation of TRPV4 channels located in the smooth muscle cell membrane. Calcium entry via the activated TRPV4 channels then appears to activate the release of calcium from the sarco-endoplasmic reticulum in the form of local calcium release events termed calcium sparks. EET-generated Ca2+ sparks then activate nearby sarcolemmal large conductance Ca2+-sensitive K+ (BKCa) channels and increase the frequency of transient K+ currents (referred to as “spontaneous transient outward currents” or STOCs). The EET-induced increases in STOC frequency results in smooth muscle hyperpolarization and vasodilation. The conclusion of this work is that TRPV4 forms a novel Ca2+-signaling complex with ryanodine receptors and BKCa channels that elicits smooth muscle hyperpolarization and arterial dilation via Ca2+-induced Ca2+ release in response to an endothelial-derived factor (see Figure 26.1).
Pressure-Induced TRP Channel Activation in Vascular Smooth Muscle
Resistance arteries constrict in response to increasing intraluminal pressure [37]. This phenomenon, known as the vascular myogenic response, is an important autoregulatory mechanism that allows blood flow to remain relatively constant despite large changes in intravascular pressure [38]. Myogenic constriction results from activation of voltage-dependent Ca2+ channels [39] secondary to smooth muscle cell membrane depolarization [40]. Activation of mechanosensitive ion channels appears to play a central role in pressure-induced depolarization and myogenic constriction. Consistent with this hypothesis, a number of early reports describe Gd3+-sensitive stretch-activated nonselective cation channels in smooth muscle cells. These stretch-activated channels have properties that are similar to those of expressed TRPs, leading some investigators to examine potential roles for TRP channels in myogenic constriction. For example, Welsh et al. [33] demonstrated a role for TRPC6 in pressure-induced smooth muscle depolarization and vasoconstriction of rat cerebral resistance arteries. In this study, cerebral myocytes were patch clamped in the whole-cell configuration. Cation currents were activated when cells were exposed to a hypotonic solution to induce membrane stretch. Downregulation of TRPC6 expression using antisense oligodeoxynucleotides attenuated swelling-activated currents in cerebral myocytes, suggesting that this channel is activated by mechanical stimulus in these cells. In isolated cerebral arteries, smooth muscle membrane depolarization and vasoconstriction induced by elevation of intraluminal pressure was also attenuated by TRPC6 antisense. These findings support the conclusion that membrane stretch activates a TRPC6-dependent depolarizing cation current that contributes to myogenic constriction of cerebral arteries. Although direct mechanosensitivity of the channel was not demonstrated, the authors speculate that membrane stretch activates PLC, thereby producing the second messenger DAG, which directly activates TRPC6 [41]. In support of this idea, Park et al. [42] reported that mechanosensitive channels in vascular smooth muscle with properties similar to those of TRPC channels were inhibited by PLC blockade and activated by a DAG analogue. Furthermore, Slish et al. [43] found that pressure-induced depolarization of cerebral artery myocytes was blocked by PLC inhibition.
Two recent reports suggest that TRPM4, a member of the melastatin TRP family, may also contribute to stretch-induced depolarization of cerebral artery myocytes. In a preliminary study, Morita et al. [44] reported activation of cation channels in cultured cells expressing cloned TRPM4 by applying negative pressure to the patch pipette. Similar stretch-activated channels were present in freshly isolated cerebral artery myocytes, suggesting that TRPM4 may be a mechanosensitive channel in these cells. Consistent with this proposal, Earley et al. [45] reported that antisense-mediated downregulation of TRPM4 impaired pressure-induced depolarization and myogenic constriction of isolated cerebral arteries. Thus, it appears that both TRPC6 and TRPM4 are key components of the myogenic response in cerebral vascular myocytes and may participate in the autoregulation of cerebral blood flow (see Figure 26.2).
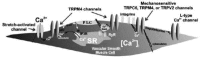
FIGURE 26.2
TRPC6, TRPM4, and TRPV2 channels are likely candidates as stretch-activated cation channels in vascular smooth muscle. These channels may be directly activated by membrane stretch or may be activated secondary to stretch activation of cell-signaling pathways (more...)
A study by Muraki et al. [46] examined the possibility that TRPV2 is a mechanically activated ion channel in smooth muscle cells isolated from mouse aortas. The authors showed that hypotonic swelling of aortic myocytes activated a Ca2+ current that was not affected by inhibitors of L-type voltage-dependent calcium channels or pretreatment with caffeine to deplete intracellular Ca2+ stores. This swelling-activated current was blocked by ruthenium red, a nonselective inhibitor of TRPV channels, and by antisense-mediated suppression of TRPV2 expression. In addition, in cultured cells expressing cloned TRPV2, currents were activated by mechanical stretch (induced by applying negative pressure to the patch pipette) as well as by hypotonic stimuli. These findings suggest a potential role for TRPV2 in pressure-induced vasoconstrictor responses, although the effects of TRPV2 antisense on myogenic constriction or changes in smooth muscle membrane potential resulting from increased intravascular pressure in resistance arteries were not reported.
Another recent report indicates that TRPC1 is a molecular candidate for the long-known mechanosensitive cation channel in oocytes [47]. TRPC1 is clearly present in vascular smooth muscle cells [48], but no evidence has yet been presented to indicate a mechanosensitive role for TRPC1 in the vasculature.
These reports suggest that multiple TRP channels expressed by vascular smooth muscle cells participate in pressure-induced depolarization and myogenic constriction of small arteries. Signaling pathways for channel activation during intraluminal pressure changes remain to be elucidated. However, it is clear that TRP channels are critical components of the myogenic response and likely play an important role in the autoregulation of blood flow (see Figure 26.2).
Store-Operated TRP Channel Activation in Vascular Smooth Muscle
In many cell types, depletion of calcium stores can trigger influx of calcium via what have been termed store-operated channels (SOCs). This type of calcium influx is thought to be important for maintaining normal function of the sarcoplasmic reticulum under a variety of conditions. The molecular identity of SOCs has been somewhat elusive, but evidence to suggest a role for TRP channels in this process is rather compelling.
Recent studies suggest that TRPC1 is an integral component of store-operated Ca2+ channels in vascular smooth muscle cells. In isolated rabbit arteriolar smooth muscle cells, Xu and Beech [48] depleted intracellular Ca2+ stores with thapsigargin in the presence of a voltage-gated Ca2+ channel blocker and “zero” extracellular Ca2+. When these cells were reintroduced to 1.5 mM extracellular Ca2+, an intracellular Ca2+ signal was recorded that was significantly reduced in cells exposed to an antibody that binds to an epitope near the proposed pore-forming region of TRPC1. Similarly, Sweeney et al. [49] reported that both calcium entry and whole-cell cation currents induced by store depletion in proliferating human pulmonary artery smooth muscle cells are significantly reduced following antisense-mediated TRPC1 down-regulation. While blockade of TRPC1 reduces store-operated Ca2+ entry, overexpression of TRPC1 reportedly has the opposite effect and increases store-operated Ca2+ entry [50]. In this study, rat pulmonary vascular rings were transfected with the human TRPC1 using an adenoviral vector. After 24 to 48 hours of organ culture, the rings were subjected to traditional protocols that elicit store-operated Ca2+ entry. Rings transfected with TRPC1 showed significantly larger intracellular Ca2+ signals and greater active tension development when Ca2+ was reintroduced to the bath solution. Likewise, upregulation of TRPC1 expression during organ culture of rat cerebral arteries is associated with a substantial increase in store-operated Ca2+ entry [34], suggesting that TRPC1 forms store-operated Ca2+ influx channels in vascular myocytes. Other studies support a role for either TRPC4 [51] or TRPC6 [52] in capacitative calcium entry and an associated proliferative response of human pulmonary artery smooth muscle cells. It appears that multiple TRPC channels may play important roles in the mechanism of calcium entry triggered in response to depletion of Ca2+ stores in vascular smooth muscle, and these Ca2+ responses may play a role in some vascular pathologies.
TRP CHANNEL FUNCTION IN VASCULAR ENDOTHELIAL CELLS
Presence of TRP Channels in the Endothelium
There is good evidence derived from both molecular and histological approaches from multiple studies showing that TRP channels are widely expressed in endothelial cells. Although the TRP channel expression pattern in the endothelium appears to vary by species, all of the TRPC channel isoforms have been detected in endothelial cells [2]. TRP channel isoforms from the two other major TRP subfamilies appear to be less abundant in endothelial cells. However, work by Fantozzi et al. [53] and Wissenbach et al. [54] illustrates that TRPM4 and TRPV4 are clearly present in endothelial cells.
TRP Channels and Endothelial Cell Calcium Entry
Most TRP channels have substantial permeability to calcium ions. This may represent a significant pathway for endothelial cell calcium influx in response to various forms of stimulation, including receptor activation and shear stress, or following depletion of calcium stores. Evidence suggests that TRPC1 channels are involved in store-operated calcium entry in human pulmonary artery endothelial cells. A study by Brough et al. [55] found that SOC entry induced by thapsigargin was decreased by about 40 percent in cultured endothelial cells treated with antisense oligonucleotides directed against TRPC1. Others have presented evidence showing that receptor activation triggers endothelial cell calcium entry, mediated at least in part by TRPC1. For instance, Antoniotti et al. [56] observed that basic fibroblast growth factor (bFGF) induced a sustained increase in cytoplasmic calcium levels in cultured bovine aortic endothelial cells. Addition of a polyclonal antibody against TRPC1 caused a 40 percent reversal of the bFGF-induced calcium increase, suggesting that the calcium influx occurred through TRPC1 channels. In human mesenteric artery endothelial cells that prominently express TRPC1 channels, bradykinin activates a gadolinium-sensitive cation channel [57] that represents a potential route for calcium entry. Although the molecular identity of the channel was not established in this study, its properties resemble those of expressed TRPC1 channels.
Heterologously expressed TRPC3 channels are known to form both receptor-and store-operated calcium channels [58]. This also appears to be true for TRPC3 channels expressed by endothelial cells. Depletion of calcium stores in human umbilical vein endothelial cells induces a cation current that is abolished in cells expressing an N-terminal, dominant negative fragment of TRPC3 [59]. When human TRPC3 channels are heterologously expressed in bovine pulmonary endothelial cells, they are not activated by depletion of calcium stores but are activated by receptor ligands such as ATP and bradykinin [60]. These findings clearly implicate TRPC3 as both an SOC entry pathway as well as a receptor-operated calcium entry channel and suggest that TRPC3 channels are centrally involved in endothelium-dependent regulation of vascular function (see Figure 26.3).
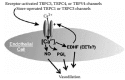
FIGURE 26.3
Endothelial TRP channels may contribute to the regulation of calcium entry and activity of endothelial vasodilator factors.
TRPC4 knockout mice have provided a powerful tool for demonstrating functional roles for this channel. Studies using these mice suggest that TRPC4 channels expressed by endothelial cells appear to be important in store-operated and agonist-induced calcium entry and endothelium-dependent vasodilation. Store-operated calcium currents in aortic endothelial cells are abolished in TRPC4 knockout mice [61]. Further, agonist-induced endothelial calcium entry and endothelium-dependent vasodilation are also greatly suppressed in these mice. Similarly, calcium entry induced by thrombin or by activation of the PAR-1 receptor in pulmonary endothelial cells is absent in TRPC4 knockout mice [62]. It has been suggested that activation of store-operated, endothelial cell calcium entry via TRPC4 channels is regulated through physical interactions of TRPC4 channels with the actin cytoskeleton, perhaps as part of a larger protein complex that could include TRPC1 as well [63].
Endothelial cell cation channels having properties very similar to those of cloned TRPV4 channels are activated by 5,6 EET [64,65], a putative endothelium-derived hyper-polarizing factor [66]. Channel activation by 5,6 EET is accompanied by a substantial increase in endothelial cell calcium, implying that endothelial TRPV4 channels may contribute to the vasodilator effects of endogenous arachidonic acid metabolites. Thus TRPV4 channels located both in endothelial cells and in smooth muscle (see above) appear to contribute to the vasodilator response to EETs.
TRP Channels in the Proliferative Response of the Endothelium to Hypoxia
Endothelial cells proliferate in response to hypoxic exposure. Fantozzi et al. [53] have presented evidence suggesting a role for endothelial TRP channels in this proliferative response in human pulmonary artery endothelial cells. Proliferation is associated with an increase in the expression of AP-1 receptors, elevated store-operated calcium entry, and enhanced TRPC4 channel expression. The increase in AP-1 receptor number following hypoxic exposure is greatly reduced in endothelial cells treated with TRPC antisense oligonucleotides, suggesting a linkage between TRPC4 channels and the proliferative response of pulmonary endothelial cells to hypoxia.
TRP Channels and Regulation of Capillary Permeability
A key role for TRPC4 channels in the regulation of endothelial capillary permeability has been recently proposed [67]. Activation of thrombin receptors or the proteinase-activated receptor-1 (PAR-1) in wild-type pulmonary endothelial cells induces a lanthanum-sensitive calcium influx and an associated increase in endothelial capillary permeability [62]. These responses were reduced by about 50 percent in endothelial cells from TRPC4 knockout versus wild-type mice, suggesting that TRPC4 channels are critical mediators of thrombin and PAR-1-induced Ca2+ influx.
Role of TRP Channels in Endothelial Cell Response to Temperature Change
TRPV channels are involved in cellular responses to environmental stimuli such as changes in osmolarity and temperature [4]. TRPV4 channels are highly expressed in endothelial cells, and a recent study suggests that these channels may be involved in regulation of vascular function in response to changes in ambient temperature [68]. These findings demonstrate that the properties of native thermosensitive cation channels in aortic endothelial cells were indistinguishable from those of heterologously expressed TRPV4 channels. The authors propose that altered calcium influx through TRPV4 at different temperatures could modulate nitric oxide production to induce vasoconstriction at lower temperatures and vasodilation at higher temperatures.
TRP Channels as Sensors of Endothelial Oxidative Stress
TRPC3 channels may be involved in the endothelial cell response to oxidative stress [69]. One study reports that oxidative stress of cultured porcine aortic endothelial cells activates a nonselective cation current and depolarizes the endothelial cells. The oxidant-induced current is abolished in cells expressing an N-terminal, dominant negative TRPC3 fragment, clearly implicating TRPC3 in the response.
SUMMARY AND FUTURE PERSPECTIVES
Growing evidence demonstrates that TRP channel proteins form functional nonselective cation channels in vascular smooth muscle and endothelial cells (Table 26.1). These channels appear to be activated by various stimuli including mechanical perturbations, receptor activation, calcium store depletion, or changes in temperature, and the channels may be involved in multiple cellular processes in the vasculature. The specific roles of these channels in normal and pathologically impaired vascular function are only just beginning to come to light but include neurotransmission, hormonal control of the vasculature, nitric oxide production, myogenic tone and autoregulation of blood flow, thermoregulation, responses to oxidative stress, and cellular proliferative activity. Further research toward understanding the interactions of TRP channels with other proteins and signaling mechanisms is clearly needed in order to understand the functional significance of TRP channels in vascular smooth muscle and endothelial cells.
REFERENCES
- 1.
- Albert AP, Large WA. Signal transduction pathways and gating mechanisms of native TRP-like cation channels in vascular myocytes. J Physiol. 2005;570:45–51. [PMC free article: PMC1464290] [PubMed: 16195316]
- 2.
- Yao X, Garland CJ. Recent developments in vascular endothelial cell transient receptor potential channels. Circ Res. 2005;97(9):853–63. [PubMed: 16254217]
- 3.
- Beech DJ, Muraki K, Flemming R. Non-selective cationic channels of smooth muscle and the mammalian homologues of Drosophila TRP. J Physiol. 2004;559(Pt. 3):685–706. [PMC free article: PMC1665181] [PubMed: 15272031]
- 4.
- Clapham DE. TRP channels as cellular sensors. Nature. 2003;426(6966):517–24. [PubMed: 14654832]
- 5.
- Xu XZ, Li HS, Guggino WB, Montell C. Coassembly of TRP and TRPL produces a distinct store-operated conductance. Cell. 1997;89(7):1155–64. [PubMed: 9215637]
- 6.
- Hofmann T, Schaefer M, Schultz G, Gudermann T. Subunit composition of mammalian transient receptor potential channels in living cells. Proc Natl Acad Sci USA. 2002;99(11):7461–66. [PMC free article: PMC124253] [PubMed: 12032305]
- 7.
- Strubing C, Krapivinsky G, Krapivinsky L, Clapham DE. Formation of novel TRPC channels by complex subunit interactions in embryonic brain. J Biol Chem. 2003;278(40):39014–19. [PubMed: 12857742]
- 8.
- Strubing C, Krapivinsky G, Krapivinsky L, Clapham DE. TRPC1 and TRPC5 form a novel cation channel in mammalian brain. Neuron. 2001;29(3):645–55. [PubMed: 11301024]
- 9.
- Walker RL, Hume JR, Horowitz B. Differential expression and alternative splicing of TRP channel genes in smooth muscles. Am J Physiol Cell Physiol. 2001;280(5):C1184–92. [PubMed: 11287332]
- 10.
- Lee MW, Severson DL. Signal transduction in vascular smooth muscle: diacylglycerol second messengers and PKC action. Am J Physiol. 1994;267(3 Pt. 1):C659–78. [PubMed: 7943196]
- 11.
- Berridge MJ, Lipp P, Bootman MD. The versatility and universality of calcium signalling. Nat Rev Mol Cell Biol. 2000;1(1):11–21. [PubMed: 11413485]
- 12.
- Putney JW Jr. A model for receptor-regulated calcium entry. Cell Calcium. 1986;7(1):1–12. [PubMed: 2420465]
- 13.
- Chen XL, Rembold CM. Phenylephrine contracts rat tail artery by one electromechanical and three pharmacomechanical mechanisms. Am J Physiol. 1995;268(1 Pt. 2):H74–81. [PubMed: 7840305]
- 14.
- Nelson MT, Patlak JB, Worley JF, Standen NB. Calcium channels, potassium channels, and voltage dependence of arterial smooth muscle tone. Am J Physiol. 1990;259(1 Pt. 1):C3–18. [PubMed: 2164782]
- 15.
- Karaki H, Ozaki H, Hori M, Mitsui-Saito M, Amano K, Harada K, Miyamoto S, Nakazawa H, Won KJ, Sato K. Calcium movements, distribution, and functions in smooth muscle. Pharmacol Rev. 1997;49(2):157–230. [PubMed: 9228665]
- 16.
- Byrne NG, Large WA. Membrane ionic mechanisms activated by noradrenaline in cells isolated from the rabbit portal vein. J Physiol. 1988;404:557–73. [PMC free article: PMC1190842] [PubMed: 2473199]
- 17.
- Chen C, Wagoner PK. Endothelin induces a nonselective cation current in vascular smooth muscle cells. Circ Res. 1991;69(2):447–54. [PubMed: 1713535]
- 18.
- Helliwell RM, Large WA. Alpha 1-adrenoceptor activation of a non-selective cation current in rabbit portal vein by 1,2-diacyl-sn-glycerol. J Physiol. 1997;499(Pt. 2):417–28. [PMC free article: PMC1159316] [PubMed: 9080371]
- 19.
- Nakajima T, Hazama H, Hamada E, Wu SN, Igarashi K, Yamashita T, Seyama Y, Omata M, Kurachi Y. Endothelin-1 and vasopressin activate Ca(2+)-permeable non-selective cation channels in aortic smooth muscle cells: mechanism of receptor-mediated Ca2+ influx. J Mol Cell Cardiol. 1996;28(4):707–22. [PubMed: 8732499]
- 20.
- Montell C. TRP trapped in fly signaling web. Curr Opin Neurobiol. 1998;8(3):389–97. [PubMed: 9687357]
- 21.
- Hardie RC, Minke B. Phosphoinositide-mediated phototransduction in Drosophila photoreceptors: the role of Ca2+ and trp. Cell Calcium. 1995;18(4):256–74. [PubMed: 8556766]
- 22.
- Yu Y, Fantozzi I, Remillard CV, Landsberg JW, Kunichika N, Platoshyn O, Tigno DD, Thistlethwaite PA, Rubin LJ, Yuan JX. Enhanced expression of transient receptor potential channels in idiopathic pulmonary arterial hypertension. Proc Natl Acad Sci USA. 2004;101(38):13861–66. [PMC free article: PMC518765] [PubMed: 15358862]
- 23.
- McDaniel SS, Platoshyn O, Wang J, Yu Y, Sweeney M, Krick S, Rubin LJ, Yuan JX. Capacitative Ca(2+) entry in agonist-induced pulmonary vasoconstriction. Am J Physiol Lung Cell Mol Physiol. 2001;280(5):L870–880. [PubMed: 11290510]
- 24.
- Clapham DE, Runnels LW, Strubing C. The TRP ion channel family. Nat Rev Neurosci. 2001;2(6):387–96. [PubMed: 11389472]
- 25.
- Harteneck C, Plant TD, Schultz G. From worm to man: three subfamilies of TRP channels. Trends Neurosci. 2000;23(4):159–66. [PubMed: 10717675]
- 26.
- Lintschinger B, Balzer-Geldsetzer M, Baskaran T, Graier WF, Romanin C, Zhu MX, Groschner K. Coassembly of Trp1 and Trp3 proteins generates diacylglycerol- and Ca2+-sensitive cation channels. J Biol Chem. 2000;275(36):27799–805. [PubMed: 10882720]
- 27.
- Wu X, Babnigg G, Villereal ML. Functional significance of human trp1 and trp3 in store-operated Ca(2+) entry in HEK-293 cells. Am J Physiol Cell Physiol. 2000;278(3):C526–36. [PubMed: 10712241]
- 28.
- Inoue R, Okada T, Onoue H, Hara Y, Shimizu S, Naitoh S, Ito Y, Mori Y. The transient receptor potential protein homologue TRP6 is the essential component of vascular alpha(1)-adrenoceptor-activated Ca(2+)-permeable cation channel. Circ Res. 2001;88(3):325–32. [PubMed: 11179201]
- 29.
- Jung S, Strotmann R, Schultz G, Plant TD. TRPC6 is a candidate channel involved in receptor-stimulated cation currents in A7r5 smooth muscle cells. Am J Physiol Cell Physiol. 2002;282(2):C347–59. [PubMed: 11788346]
- 30.
- Welsh DG, Brayden JE. Mechanisms of coronary artery depolarization by uridine triphosphate. Am J Physiol Heart Circ Physiol. 2001;280(6):H2545–53. [PubMed: 11356609]
- 31.
- Reading SA, Earley S, Waldron BJ, Welsh DG, Brayden JE. TRPC3 mediates pyrimidine receptor-induced depolarization of cerebral arteries. Am J Physiol Heart Circ Physiol. 2005;288(5):H2055–61. [PubMed: 15604128]
- 32.
- Reading SA, Lutz KE, Brayden JE. Ca2+ influx through TRPC3 channels contributes to agonist-induced constriction of cerebral arteries. FASEB J. 2005;19(5):A1625.
- 33.
- Welsh DG, Morielli AD, Nelson MT, Brayden JE. Transient receptor potential channels regulate myogenic tone of resistance arteries. Circ Res. 2002;90(3):248–50. [PubMed: 11861411]
- 34.
- Bergdahl A, Gomez MF, Wihlborg AK, Erlinge D, Eyjolfson A, Xu SZ, Beech DJ, Dreja K, Hellstrand P. Plasticity of TRPC expression in arterial smooth muscle: correlation with store-operated Ca2+ entry. Am J Physiol Cell Physiol. 2005;288(4):C872–80. [PubMed: 15561760]
- 35.
- Lin MJ, Leung GP, Zhang WM, Yang XR, Yip KP, Tse CM, Sham JS. Chronic hypoxia-induced upregulation of store-operated and receptor-operated Ca2+ channels in pulmonary arterial smooth muscle cells: a novel mechanism of hypoxic pulmonary hypertension. Circ Res. 2004;95(5):496–505. [PubMed: 15256480]
- 36.
- Earley S, Heppner TJ, Nelson MT, Brayden JE. TRPV4 forms a novel Ca2+ signaling complex with ryanodine receptors and BKCa channels. Circ Res. 2005;97(12):1270–79. [PubMed: 16269659]
- 37.
- Bayliss WM. On the local reactions of the arterial wall to changes of internal pressure. J Physiol (London). 1902;28:220–31. [PMC free article: PMC1540533] [PubMed: 16992618]
- 38.
- Davis MJ, Hill MA. Signaling mechanisms underlying the vascular myogenic response. Physiol Rev. 1999;79(2):387–423. [PubMed: 10221985]
- 39.
- Knot HJ, Nelson MT. Regulation of arterial diameter and wall [Ca2+] in cerebral arteries of rat by membrane potential and intravascular pressure. J Physiol. 1998;508(Pt. 1):199–209. [PMC free article: PMC2230857] [PubMed: 9490839]
- 40.
- Harder DR. Pressure-dependent membrane depolarization in cat middle cerebral artery. Circ Res. 1984;55(2):197–202. [PubMed: 6744529]
- 41.
- Hofmann T, Obukhov AG, Schaefer M, Harteneck C, Gudermann T, Schultz G. Direct activation of human TRPC6 and TRPC3 channels by diacylglycerol. Nature. 1999;397(6716):259–63. [PubMed: 9930701]
- 42.
- Park KS, Kim Y, Lee YH, Earm YE, Ho WK. Mechanosensitive cation channels in arterial smooth muscle cells are activated by diacylglycerol and inhibited by phospholipase C inhibitor. Circ Res. 2003;93(6):557–64. [PubMed: 12946944]
- 43.
- Slish DF, Welsh DG, Brayden JE. Diacylglycerol and protein kinase C activate cation channels involved in myogenic tone. Am J Physiol Heart Circ Physiol. 2002;283(6):H2196–201. [PubMed: 12388226]
- 44.
- Morita H, Honda A, Nelson MT, Brayden JE. Stretch activates Ca2+-sensitive, non-selective cation channels in smooth muscle cells from cerebral arteries. FASEB J. 2003;17(4):A64. (Abstract)
- 45.
- Earley S, Waldron BJ, Brayden JE. Critical role for transient receptor potential channel TRPM4 in myogenic constriction of cerebral arteries. Circ Res. 2004;95(9):922–29. [PubMed: 15472118]
- 46.
- Muraki K, Iwata Y, Katanosaka Y, Ito T, Ohya S, Shigekawa M, Imaizumi Y. TRPV2 is a component of osmotically sensitive cation channels in murine aortic myocytes. Circ Res. 2003;93(9):829–38. [PubMed: 14512441]
- 47.
- Maroto R, Raso A, Wood TG, Kurosky A, Martinac B, Hamill OP. TRPC1 forms the stretch-activated cation channel in vertebrate cells. Nat Cell Biol. 2005;7(2):179–85. [PubMed: 15665854]
- 48.
- Xu SZ, Beech DJ. TrpC1 is a membrane-spanning subunit of store-operated Ca(2+) channels in native vascular smooth muscle cells. Circ Res. 2001;88(1):84–87. [PubMed: 11139478]
- 49.
- Sweeney M, Yu Y, Platoshyn O, Zhang S, McDaniel SS, Yuan JX. Inhibition of endogenous TRP1 decreases capacitative Ca2+ entry and attenuates pulmonary artery smooth muscle cell proliferation. Am J Physiol Lung Cell Mol Physiol. 2002;283(1):L144–55. [PubMed: 12060571]
- 50.
- Kunichika N, Yu Y, Remillard CV, Platoshyn O, Zhang S, Yuan JX. Overexpression of TRPC1 enhances pulmonary vasoconstriction induced by capacitative Ca2+ entry. Am J Physiol Lung Cell Mol Physiol. 2004;287(5):L962–99. [PubMed: 15220115]
- 51.
- Zhang S, Remillard CV, Fantozzi I, Yuan JX. ATP-induced mitogenesis is mediated by cyclic AMP response element-binding protein-enhanced TRPC4 expression and activity in human pulmonary artery smooth muscle cells. Am J Physiol Cell Physiol. 2004;287(5):C1192–201. [PubMed: 15229105]
- 52.
- Yu Y, Sweeney M, Zhang S, Platoshyn O, Landsberg J, Rothman A, Yuan JX. PDGF stimulates pulmonary vascular smooth muscle cell proliferation by upregulating TRPC6 expression. Am J Physiol Cell Physiol. 2003;284(2):C316–30. [PubMed: 12529250]
- 53.
- Fantozzi I, Zhang S, Platoshyn O, Remillard CV, Cowling RT, Yuan JX. Hypoxia increases AP-1 binding activity by enhancing capacitative Ca2+ entry in human pulmonary artery endothelial cells. Am J Physiol Lung Cell Mol Physiol. 2003;285(6):L1233–45. [PubMed: 12909593]
- 54.
- Wissenbach U, Bodding M, Freichel M, Flockerzi V. Trp12, a novel Trp-related protein from kidney. FEBS Lett. 2000;485(2-3):127–34. [PubMed: 11094154]
- 55.
- Brough GH, Wu S, Cioffi D, Moore TM, Li M, Dean N, Stevens T. Contribution of endogenously expressed Trp1 to a Ca2+-selective, store-operated Ca2+ entry pathway. FASEB J. 2001;15(10):1727–38. [PubMed: 11481220]
- 56.
- Antoniotti S, Lovisolo D, Fiorio Pla A, Munaron L. Expression and functional role of bTRPC1 channels in native endothelial cells. FEBS Lett. 2002;510(3):189–95. [PubMed: 11801252]
- 57.
- Kohler R, Brakemeier S, Kuhn M, Degenhardt C, Buhr H, Pries A, Hoyer J. Expression of ryanodine receptor type 3 and TRP channels in endothelial cells: comparison of in situ and cultured human endothelial cells. Cardiovasc Res. 2001;51(1):160–68. [PubMed: 11399258]
- 58.
- Venkatachalam K, van Rossum DB, Patterson RL, Ma HT, Gill DL. The cellular and molecular basis of store-operated calcium entry. Nat Cell Biol. 2002;4(11):E263–72. [PubMed: 12415286]
- 59.
- Groschner K, Hingel S, Lintschinger B, Balzer M, Romanin C, Zhu X, Schreibmayer W. Trp proteins form store-operated cation channels in human vascular endothelial cells. FEBS Lett. 1998;437(1-2):101–6. [PubMed: 9804180]
- 60.
- Kamouchi M, Philipp S, Flockerzi V, Wissenbach U, Mamin A, Raeymaekers L, Eggermont J, Droogmans G, Nilius B. Properties of heterologously expressed hTRP3 channels in bovine pulmonary artery endothelial cells. J Physiol. 1999;518(Pt. 2):345–58. [PMC free article: PMC2269435] [PubMed: 10381584]
- 61.
- Freichel M, Suh SH, Pfeifer A, Schweig U, Trost C, Weissgerber P, Biel M, Philipp S, Freise D, Droogmans G, Hofmann F, Flockerzi V, Nilius B. Lack of an endothelial store-operated Ca2+ current impairs agonist-dependent vasorelaxation in TRP4−/− mice. Nat Cell Biol. 2001;3(2):121–27. [PubMed: 11175743]
- 62.
- Tiruppathi C, Freichel M, Vogel SM, Paria BC, Mehta D, Flockerzi V, Malik AB. Impairment of store-operated Ca2+ entry in TRPC4(−/−) mice interferes with increase in lung microvascular permeability. Circ Res. 2002;91(1):70–76. [PubMed: 12114324]
- 63.
- Cioffi DL, Wu S, Stevens T. On the endothelial cell I(SOC). Cell Calcium. 2003;33(5-6):323–36. [PubMed: 12765679]
- 64.
- Watanabe H, Vriens J, Prenen J, Droogmans G, Voets T, Nilius B. Anandamide and arachidonic acid use epoxyeicosatrienoic acids to activate TRPV4 channels. Nature. 2003;424(6947):434–38. [PubMed: 12879072]
- 65.
- Vriens J, Owsianik G, Fisslthaler B, Suzuki M, Janssens A, Voets T, Morisseau C, Hammock BD, Fleming I, Busse R, Nilius B. Modulation of the Ca2+ permeable cation channel TRPV4 by cytochrome P450 epoxygenases in vascular endothelium. Circ Res. 2005;97(9):908–15. [PubMed: 16179585]
- 66.
- Campbell WB, Harder DR. Endothelium-derived hyperpolarizing factors and vascular cytochrome P450 metabolites of arachidonic acid in the regulation of tone. Circ Res. 1999;84(4):484–88. [PubMed: 10066684]
- 67.
- Tiruppathi C, Minshall RD, Paria BC, Vogel SM, Malik AB. Role of Ca2+ signaling in the regulation of endothelial permeability. Vascul Pharmacol. 2002;39(4-5):173–85. [PubMed: 12747958]
- 68.
- Watanabe H, Vriens J, Suh SH, Benham CD, Droogmans G, Nilius B. Heat-evoked activation of TRPV4 channels in a HEK293 cell expression system and in native mouse aorta endothelial cells. J Biol Chem. 2002;277(49):47044–51. [PubMed: 12354759]
- 69.
- Balzer M, Lintschinger B, Groschner K. Evidence for a role of Trp proteins in the oxidative stress-induced membrane conductances of porcine aortic endothelial cells. Cardiovasc Res. 1999;42(2):543–49. [PubMed: 10533589]
- 70.
- Yip H, Chan WY, Leung PC, Kwan HY, Liu C, Huang Y, Michel V, Yew DT, Yao X. Expression of TRPC homologs in endothelial cells and smooth muscle layers of human arteries. Histochem Cell Biol. 2004;122(6):553–61. [PubMed: 15538613]
- 71.
- Facemire CS, Mohler PJ, Arendshorst WJ. Expression and relative abundance of short transient receptor potential channels in the rat renal microcirculation. Am J Physiol Renal Physiol. 2004;286(3):F546–51. [PubMed: 14678949]
- *
Present affiliation: Colorado State University, Fort Collins.
- Review TRP Channels and Pain.[Neurobiology of TRP Channels. ...]Review TRP Channels and Pain.González-Ramírez R, Chen Y, Liedtke WB, Morales-Lázaro SL. Neurobiology of TRP Channels. 2017
- Review Muscling in on TRP channels in vascular smooth muscle cells and cardiomyocytes.[Cell Calcium. 2017]Review Muscling in on TRP channels in vascular smooth muscle cells and cardiomyocytes.Alonso-Carbajo L, Kecskes M, Jacobs G, Pironet A, Syam N, Talavera K, Vennekens R. Cell Calcium. 2017 Sep; 66:48-61. Epub 2017 Jun 15.
- Review TRP Channels: What Do They Look Like?[Neurobiology of TRP Channels. ...]Review TRP Channels: What Do They Look Like?Rosasco MG, Gordon SE. Neurobiology of TRP Channels. 2017
- Review Determining the Crystal Structure of TRPV6.[Calcium Entry Channels in Non-...]Review Determining the Crystal Structure of TRPV6.Saotome K, Singh AK, Sobolevsky AI. Calcium Entry Channels in Non-Excitable Cells. 2018
- Review TRP channels and the control of vascular function.[Curr Opin Pharmacol. 2010]Review TRP channels and the control of vascular function.Di A, Malik AB. Curr Opin Pharmacol. 2010 Apr; 10(2):127-32. Epub 2010 Jan 7.
- Functional Significance of Transient Receptor Potential Channels in Vascular Fun...Functional Significance of Transient Receptor Potential Channels in Vascular Function - TRP Ion Channel Function in Sensory Transduction and Cellular Signaling Cascades
- TRP Channel Functioning in Mating and Fertilization - TRP Ion Channel Function i...TRP Channel Functioning in Mating and Fertilization - TRP Ion Channel Function in Sensory Transduction and Cellular Signaling Cascades
- Voltage and Temperature Gating of ThermoTRP Channels - TRP Ion Channel Function ...Voltage and Temperature Gating of ThermoTRP Channels - TRP Ion Channel Function in Sensory Transduction and Cellular Signaling Cascades
Your browsing activity is empty.
Activity recording is turned off.
See more...