NCBI Bookshelf. A service of the National Library of Medicine, National Institutes of Health.
Liedtke WB, Heller S, editors. TRP Ion Channel Function in Sensory Transduction and Cellular Signaling Cascades. Boca Raton (FL): CRC Press/Taylor & Francis; 2007.
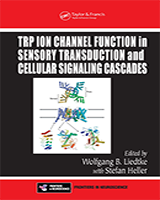
TRP Ion Channel Function in Sensory Transduction and Cellular Signaling Cascades.
Show detailsAbstract
Transient receptor potential channels (TRP) are a large family of cationic channels that are permeable to Ca2+allowing these channels to participate in a wide range of physiological processes that require Ca2+ signaling. In recent years, TRP channels have been found to play a role in many processes in the nervous system, such as the transduction of sensory stimulation [1], neuronal cell death [2], proliferation and differentiation of neural progenitor cells [3,4], nerve growth [5], and synaptic transmission [6–9]. One interesting recent discovery shows that TRPC (canonical) channels are involved in the signal transduction of axon guidance during brain development.
OVERVIEW OF NERVE PATHFINDING
As the most complicated organ in animals, the brain is composed of highly ordered cell architecture and precisely wired neural circuits, which are essential for the sophisticated functions of the brain. The development of functional neuronal circuits requires the finely tuned growth of nerve fibers toward their targets, a process called nerve pathfinding [10]. Both the axon and dendrites of neurons have the pathfinding process during development, and some mechanisms were shared by these two different neuronal processes [11,12]. Therefore, in this chapter when we talk about the nerve growth or nerve guidance, we do not discuss these two processes separately without special mention. At the tip of growing nerves there is a highly dynamic structure called a growth cone, which bears motile filopodia and lamellipodia, exploring the environment and leading the nerve growth toward its favorite direction. During brain development, it is generally believed that concentration gradients of the guidance cues exist in the tissue [13,14]. When the growth cone is exposed to a concentration gradient of a chemoattractant or chemorepellent, it responds by turning toward or away from the source of the guidance cue, one of the basic behaviors of growth cones during nerve pathfinding. During this response, receptors on the growth cone’s surface can read the concentration gradient and transduce the direction signal into the cytosol, initiating a series of downstream events, including the rearrangement of the actin and microtubule cytoskeletons inside the cell [15], the changing of adhesion level at different sides of the growth cone [16], polarized endocytosis and exocytosis of vesicles containing membrane proteins, synthesis and insertion of some new proteins for nerve pathfinding [17–19], and degradation of some proteins to control the local protein level [20]. Transduction of some long-range signals back to the soma and other neurites in the same cell may be also required for the neuron to coordinate the response of different neurites in response to the stimuli [21–23]. Eventually, the growth cone will turn and grow stably in the new direction.
CALCIUM SIGNAL IN GROWTH CONE TURNING
With regard to the complicated signal transduction process during this growth cone turning triggered by guidance cues, one fundamental question is what kind of intracellular signal can be activated to mediate the directional information after the growth cone reads the concentration gradient of extracellular guidance cues. The most attractive candidate for this mediator of the growth cone guidance signal is the intracellular Ca2+ ([Ca2+]i) [24], which regulates the motility of the growth cone and the response of growth cones to many extracellular factors [25–27]. Using an in vitro growth cone guidance model [28], previous studies showed that many extracellular factors that can guide the nerve growth, including netrin-1, slit, brain-derived neurotrophic factor (BDNF), myelin-associated glycoprotein (MAG), SDF-1, and several neurotranmitters [22,29–33], can elevate the [Ca2+]i in the growth cone. Preventing the [Ca2+]i elevation in growth cones by removing the extracellular Ca2+ (for some factors), by depleting the intracellular Ca2+ store, or by blocking the Ca2+ release from internal stores, inhibits the growth cone turning triggered by a concentration gradient of guidance cues [24]. A gradient of [Ca2+]i at the growth cone is also sufficient to drive the nerve growth cone turning because an artificially generated concentration gradient of [Ca2+]i, either by local photolysis of caged compound of Ca2+ or by an extracellular gradient of ryanodine (a drug that opens the internal store of Ca2+), can trigger the growth cone to extend toward the higher [Ca2+]i side [29,34]. Moreover, Ca2+ has been shown to regulate a wide range of intracellular signaling molecules involved in nerve growth, including those microtubule- and actin-associated proteins, motor proteins, kinases, phosphotases, and proteases [24]. Based on these facts, a hypothesis is widely accepted that a concentration gradient of extracellular guidance factors can be translated into a concentration gradient of [Ca2+]i across the growth cone, which then drives the preferential growth of the nerve toward the higher [Ca2+]i side.
Under such a hypothesis, it is of great interest to know how the Ca2+ signal is triggered by guidance factors. Elevation of [Ca2+]i can result from Ca2+ influx through voltage-gated Ca2+ channels in plasma membrane in response to membrane depolarization or from the opening of ligand-gated Ca2+ channels, for example, ionotropic glutamate receptors. Internal release of Ca2+ triggered by the messenger molecule inositol-1,4,5-trisphosphate (InsP3) or by Ca2+ itself may also contribute to elevation of [Ca2+]i [24]. Another important pathway that can lead to the elevation of [Ca2+]i is the Ca2+ influx through the voltage-independent channels including the TRP channels, which may be gated by both receptor activation and store-operated mechanisms [1,35].
Previous studies showed that both the Ca2+ influx through membrane channels and the Ca2+ release from internal stores are required for the growth cone turning triggered by a group of guidance molecules including netrin, BDNF, and MAG [13,29,30,36]. In these studies, it was shown that netrin triggers the opening of the L-type Ca2+ channel, an ion channel that is opened upon membrane depolarization, and Ca2+ influx through this voltage-gated Ca2+ channel is required for the attractive growth cone turning triggered by netrin [29,37]. Observations from a lot of other researchers also showed that during early development spontaneous neuronal activity is required for the proper growth and nerve pathfinding through elevating the [Ca2+]i [30,38,39]. One unavoidable question raised by these studies is how could membrane potential be elevated during pathfinding of these immature neurons? This puzzle obtained an attractive answer by the exciting recent discovery that TRPC channels, whose openings are not voltage dependent, play essential roles in the axon pathfinding.
TRPCS’ FUNCTION IN NERVE GUIDANCE
TRPC5 Regulates Nerve Growth
The first clue comes from the neurite extension study carried out in cultured hippocampal neurons [5]. In order to address the function of TRPC5 channels that are expressed at high levels in the hippocampus, the dominant negative mutant proteins of TRPC5 were expressed in cultured hippocampal neurons. One interesting finding is that overexpression of wide-type TRPC5 in these neurons dramatically reduced the neurite extension rate. On the contrary, expression of dominant negative TRPC5 resulted in increased filopodia length and an elevated neurite extension rate. Consistently, a substantial amount of TRPC5 protein can be detected at the growth cone probed with a specific anti-TRPC5 antibody. The researchers concluded from these observations that spontaneous opening of TRPC5 may mediate the influx of Ca2+ from extracellular media, which may be adverse for the neurite extension. Since the TRPC5 channel regulates the growth cone morphology and the neurite extension rate, it is highly possible that these Ca2+-permeable channels may be involved in the growth cone pathfinding, which is the neurite extension controlled by extracellular factors.
TRPC Contributes to Nerve Pathfinding
New findings verified this prediction. Three independent studies showed that TRPC channels may be involved in the attractive growth cone turning triggered by the chemoattractant netrin or BDNF in cultured cerebellar granule cells of rat and embryonic spinal neurons of Xenopus [40–42]. In these studies, growth cone turning assay was used to test the response of the growth cone to an extracellular gradient of guidance factors [16,28]. A micropipette containing guidance molecules at a very high dosage was used to generate a concentration gradient of the molecule through repetitive puffing, and the gradient source (the pipette tip) was positioned 100 μm in front of the growth cone at an angle of 45 degrees with respect to the original direction of the nerve extension. The growth cone can sense this concentration gradient of guidance molecules and migrate toward or away from the gradient, depending on what guidance molecules are tested. Interestingly, all these studies showed that chemoattraction triggered by BDNF and netrin was blocked by inhibiting TRP channels with a general inhibitor SKF-96365 or by specific knocking down of the expression of endogenous TRPC protein with small interference RNA (siRNA) or morpholino-antisense RNA. Meanwhile, the [Ca2+]i elevation triggered by netrin and BDNF at the growth cone was also dramatically reduced after perturbing the TRPC channels, as revealed by Ca2+ imaging or patch-clamp recording at the growth cone. This suggests that in response to the stimulation of BDNF and netrin, TRPC channels may mediate the Ca2+ influx, which is required for triggering the growth cone turning. Moreover, the cation influx through TRP channels may elevate the level of membrane potential, leading to the activation of a voltage-dependent sodium channel and calcium channels, which further depolarize membrane potential and allow more Ca2+ influx. These studies also showed that the role of TRPC channels in growth cone turning is specific. In rat granule neurons, TRPC3 and 6 are only required for BDNF-triggered growth cone attraction but not for either growth cone attraction triggered by glutamate or repulsion triggered by SDF-1. In the Xenopus spinal neurons, the chemoattractant netrin and BDNF as well as the repellent MAG engage Xenopus TRP-1 (xTRPC1) to guide the growth cone, while another guidance molecule (semaphorin) doesn’t use it [42].
The requirement of TRPC channels in the axon guidance in vivo was further demonstrated by Shim et al. [42] Apart from a similar observation using the growth cone turning assay, they looked at the growth of the commissural axons in the spinal cord after injection of the morphalino RNA of the xTRPC1 in one cell at the two-cell stage of the embryo to silence the expression of xTRPC1 proteins during development. They found that knocking down the expression of xTRPC1 dramatically deferred the midline cross of these commissural axons and caused some axons to go astray, suggesting that the xTRPC1 channel can mediate the axon guidance signal in vivo. Although currently no TRPC knockout mice have been reported to have nerve pathfinding defects, it may be due to the compensation of protein function by other TRP family members when a single TRPC gene is knocked out. It is possible that defects in nerve pathfinding may be seen in mice of compound knockout of several family members.
Not only chemoattraction requires TRPC channels, it was also shown that the repulsive growth cone turning triggered by MAG was also eliminated by blocking the xTRPC channels [42]. Because MAG is one of the molecules that prevents axon regeneration after the injury of central nervous systems in adult animals, the engagement of the TRPC channel in MAG signaling suggests that these ion channels will be candidate drug targets to enhance nerve regeneration.
TRPC Gating Mechanisms by Guidance Factors
One study explored the opening mechanism of the TRPC channel in the growth cone by the guidance molecule BDNF [40]. Similar to the PLC dependency of many other TRP channels, the researchers found that both the growth cone attraction and the elevation of [Ca2+]i triggered by BDNF were blocked by inhibition of PLC signaling with the specific inhibitor U73122. In contrast, inhibition of PKC activity did not have any effect. Furthermore, blocking the InsP3 signaling with xestospongin C or 2APB, which perturb the InsP3 receptor at the internal store of Ca2+, also blocked the growth cone turning and [Ca2+]i elevation, suggesting that Ca2+ release from internal stores may be required for the opening of TRPC channels in the plasma membrane. Overall, these observations support such a model where the binding of BDNF with its receptor TrkB activates PLC-γ, which generates IP3 [43] (see Figure 4.1). The subsequent opening of IP3 induces the Ca2+ release from internal stores. This Ca2+ elevation can further activate the TRPC channels in the plasma membrane and allow more Ca2+ influx. In such a model, TRPC channels in the growth cone membrane should be activated through some Ca2+-dependent mechanisms (Figure 4.1). This was supported by the recent report that the activation of TRPC5 by agonists can be regulated by calmodulin and [Ca2+]i [44]. Because many extracellular factors that have been shown to regulate nerve growth can activate PLC activity through receptor tyrosine kinase or G-protein-coupled receptors that are linked to the signaling of PLC-γ and PLC-β, respectively [45,46], PLC-dependent TRP channel activation may also mediate Ca2+ signaling for other guidance factors during nerve pathfinding. However, how the activation of guidance receptors is coupled to the opening of TRPC channels, and whether there is direct association of guidance receptors with the TRPC protein like the binding of TrkB with TRPC3 [47], remains to be clarified.
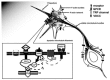
FIGURE 4.1
TRPC channels in nerve guidance. An extracellular gradient of guidance cue (from left upper corner) triggers local [Ca2+]i elevation, establishing a [Ca2+]i gradient across the growth cone. The graded calcium signal causes imbalanced polymerization/stablization (more...)
How TRPC Channels Contribute to Guidance Signaling
Among various TRPC proteins, TRPC3, TRPC6, and TRPC7 belong to one subfamily [35,48] and can form functional heteromeric or homomeric ion channels [49,50]. In cultured hippocampal neurons, expression of DN-TRPC5 elevates neurite extension, whereas overexpression of wild-type TRPC5 inhibits nerve growth [5]. However, down-regulation of TRPC3/6 did not significantly affect neurite growth in cerebellar granule cells [40]. This differential effect on nerve growth may owe to longer mean channel open time of TRPC5-containing channels than TRPC3/6 channels. That allows much larger Ca2+ influx [51–53], leading to adverse effects to neurite growth, whereas a modest level of Ca2+ influx through a TRPC3/6 channel is sufficient to trigger attractive growth cone turning. By allowing different patterns of Ca2+ influx, diverse TRPC channels may thus carry out distinct regulatory functions at the growth cone.
Previous studies have divided the guidance molecules in two groups depending on the requirement of extracellular Ca2+ and the modulation properties by cyclic AMP (cAMP) or cyclic GMP (cGMP) during the growth cone turning triggered by these factors [13]. Type I factors require the extracellular Ca2+ to trigger the growth cone turning, and cAMP level can switch the turning response of the growth cone, with a higher level of cAMP leading to chemoattraction and a lower level to repulsion. In contrast, the growth cone turning triggered by type II guidance molecules is independent of extracellular Ca2+ and is switched by intracellular cGMP level. Intracellular calcium signaling (with either type I or II factors) is required for the growth cone turning response [13]. It is possible that TRP channels participate in the growth cone guidance triggered by type I factors, by which the Ca2+ release from internal stores is not sufficient to trigger the growth cone turning. For the type II factors, however, the Ca2+ release from internal stores upon receptor activation may be enough to drive the turning responses. Meanwhile, the opening of ryanodine channels at the internal stores also contributes to the Ca2+ signaling in netrin-triggered chemoattraction [29].
For the type I guidance factors, TRPC channels may not necessarily be the sole membrane ion channels engaged in the nerve pathfinding. The opening of these cationic channels allows the depolarization of membrane potential in response to the stimulation of guidance molecules. In consequence, voltage-gated cationic channels will open and trigger the further depolarization of the membrane potential, accompanied with influx of Ca2+ through voltage-dependent calcium channels [41]. Calcium-triggered Ca2+ release from internal stores may also be triggered as a consequence of [Ca2+]i elevation. Calcium from all these sources may contribute to nerve growth and nerve pathfinding in some neuronal tissues; however, TRPC activation is the trigger of signal amplification in response to type I guidance factors.
POSSIBLE ROLES OF TRP CHANNELS IN NEURONAL MIGRATION
Calcium Signal in Neuronal Migration
Apart from nerve pathfinding, other processes in neural development may also be regulated by TRP channels. One such developmental process is neuronal migration. Unlike nerve growth, neuronal migration is the translocation of the whole cell body including the leading process and the soma. However, some signaling processes may be shared by both nerve pathfinding and neuronal migration. One example is that a series of guidance factors—slit, netrin, ephrins, BDNF, SDF—have been shown to be used for both nerve guidance and the guidance of neuronal migration [54]. Moreover, the Ca2+ signal has been shown to regulate the speed and direction of neuronal migration [55–58]. One example is the neuronal migration in postnatal cerebellum tissue, in which a high frequency of Ca2+ waves is correlated with the high migrating speed of the young granule cells [55], and the end of the Ca2+ wave is linked to the cease of the migration [58]. NMDA receptor activity and N-type Ca2+ channels are required to maintain the normal migration of these neurons [59]. Therefore, it is highly possible that TRPC channels that participate in the nerve guidance signaling, and whose openings allow Ca2+ influx into the cytosol, may also play a role in controlling the motility and the guidance of neuronal migration. Determining which TRPC proteins are involved in neuronal migration deserves further study.
Mechanosensation in Migrating Neurons?
During its migration, stress and tension are generated toward the neuron because it has to attach and squeeze in surrounding tissue, which is filled with other cells and an extracellular matrix. The same is true for the exploring growth cone, although the size of the growth cone is relatively smaller. These mechanical forces and their change over time may reflect current motility and position of the migrating cell. It is likely that cells sense and use this information to fine-tune their pace and direction of migration, such as changing their shapes in order to select a way of stronger adhesion and lower resistance to their movements. Consistent with this possibility, it was frequently observed that neurons change their shapes dramatically during migration including becoming highly elongated [60,61]. Therefore, it is reasonable to hypothesize that mechanical sensing may play an important role in neuronal migration, helping the neuron to actively reorganize the cytoskeleton, to change its shape, or even to reduce and recover its volume in order to fight its way in tissues. In agreement with this prediction, mechanically stimulated currents were recorded in the growth cones of cultured snail neurons and rat DRG neurons [62,63]. More interestingly, filopodia, which play an essential role in exploring the surrounding tissue, were the most sensitive to mechanical stimulation. Mechanical tension can be an important regulator of nerve growth because neurite outgrowth can be initiated de novo by experimental application of tension to the cell margin of neurons in culture prior to spontaneous neurite outgrowth [64–66]. The “towed” neurites had a normal appearance, showed rapid saltatory movements of internal organelles, and were capable of sustained growth on the substratum. Electron microscopy of bundles of neurites produced in this way from explanted dorsal root ganglia showed an ultrastructure typical of cultured neurites, with abundant longitudinally aligned microtubules and neurofilaments [64,67]. In cultured hippocampal neurons, applied mechanical tension could stimulate undifferentiated minor processes to become axons and could induce a second axon in an already polarized neuron [68]. In a cultured fibroblast, it was reported that stretch-activated Ca2+ entry sustains cell migration and mediates active and passive responses to mechanical signals [69]. In this study, application of gadolinium, an inhibitor of stretch-activated ion channels, or removal of extracellular-free Ca2+, caused inhibition of traction forces. Gadolinium treatment also inhibited cell migration [69]. Immunofluorescence microscopy indicated that gadolinium caused a dramatic decrease in vinculin and phosphotyrosine concentrations at focal adhesions, suggesting that stretch-activated Ca2+ entry regulates the organization of focal adhesions and the output of mechanical forces. Likewise, mechanosensory channels may be involved in the migration of neurons during development.
So far, the molecular nature for these mechanical sensors at the migrating growth cone and soma remains unknown. Some researchers reported that the N-type calcium channel on the cell membrane is mechanosensitive [70]. Interestingly, N-type calcium channels have been reported to play a role in directed migration of immature neurons as indicated by the reductive effect of the channel’s inhibitor on the migration of cultured cerebellar granule cells [71]. In contrast, inhibitors of L- and T-type calcium channels, as well as those of sodium and potassium channels, had no effect on the rate of granule cell migration [71]. Because gadolinium, a drug previously used to inhibit stretch-activated ion channels, can also block or activate TRP channels at different concentrations [72], it is unclear whether this drug reduced the migration of fibroblasts [69] through inhibiting TRP channels. The recent discovery that TRP channels play essential roles in mechanical sensing in sensory neurons [1,73] raised the possibility that these TRP channels may play a role in sensing the adhesion and compactness of the surrounding tissue of migrating neurons. Whether the mechanosensitive TRP channels in sensory neurons are also expressed in migrating neurons during development and whether they really serve as the mechanical sensor and influence neuronal migration will be fascinating subjects for further investigation.
Thermosensation for Migration?
Imaging studies in brain slice cultures showed that neuronal migration is highly sensitive to temperature [59,60,74]. Considering that TRP channels play essential roles in heat and cold sensing [1,35] and that some of these TRP channels are also expressed in the brain [35,75], one reasonable supposition is that these channels also sense temperature when the neurons migrate in a normal tissue environment, allowing Ca2+influx and maintaining a suitable level of Ca2+ in the cytosol to keep the motility of the cells. Exploring the expression patterns of TRP family members in the brain at different developmental stages will help to determine which TRP proteins may be actively involved in specific developmental processes.
CONCLUSION
Evidence is emerging that Ca2+-permeable TRP channels regulate nerve growth and pathfinding. The Ca2+ permeability and various gating mechanisms suggest that TRP channels may be widely involved in other developmental processes including neuronal migration, dendrite arborization, spine generation, and synapse formation. How TRP channels are opened by various physiological stimuli during development remains unclear. The next several years will witness the identification of more functions played by TRP channels in various developmental processes. The further clarification of the function of TRP channels and the underlying mechanisms in neural development will dramatically increase our understanding of how brain circuits are connected.
ACKNOWLEDGMENTS
We would like to thank Dr. Yi-zheng Wang, Ning Li, and Chen-bing Guan for comments on the manuscript.
REFERENCES
- 1.
- Moran MM, Xu H, Clapham DE. TRP ion channels in the nervous system. Curr Opin Neurobiol. 2004;14:362–69. [PubMed: 15194117]
- 2.
- Aarts MM, Tymianski M. TRPMs and neuronal cell death. Pflugers Arch. 2005 [PubMed: 16044308]
- 3.
- Pla AF, et al. Canonical transient receptor potential 1 plays a role in basic fibroblast growth factor (bFGF)/FGF receptor-1-induced Ca2+ entry and embryonic rat neural stem cell proliferation. J Neurosci. 2005;25:2687–701. [PMC free article: PMC6725156] [PubMed: 15758179]
- 4.
- Wu X, Zagranichnaya TK, Gurda GT, Eves EM, Villereal ML. A TRPC1/TRPC3-mediated increase in store-operated calcium entry is required for differentiation of H19-7 hippocampal neuronal cells. J Biol Chem. 2004;279:43392–402. [PubMed: 15297455]
- 5.
- Greka A, Navarro B, Oancea E, Duggan A, Clapham DE. TRPC5 is a regulator of hippocampal neurite length and growth cone morphology. Nat Neurosci. 2003;6:837–45. [PubMed: 12858178]
- 6.
- Gee CE, Benquet P, Gerber U. Group I metabotropic glutamate receptors activate a calcium-sensitive transient receptor potential-like conductance in rat hippocampus. J Physiol. 2003;546:655–64. [PMC free article: PMC2342598] [PubMed: 12562994]
- 7.
- Tozzi A, et al. Involvement of transient receptor potential-like channels in responses to mGluR-I activation in midbrain dopamine neurons. Eur J Neurosci. 2003;18:2133–145. [PubMed: 14622174]
- 8.
- Bengtson CP, Tozzi A, Bernardi G, Mercuri NB. Transient receptor potential-like channels mediate metabotropic glutamate receptor EPSCs in rat dopamine neurones. J Physiol. 2004;555:323–30. [PMC free article: PMC1664846] [PubMed: 14724196]
- 9.
- Munsch T, Freichel M, Flockerzi V, Pape HC. Contribution of transient receptor potential channels to the control of GABA release from dendrites. Proc Natl Acad Sci USA. 2003;100:16065–70. [PMC free article: PMC307693] [PubMed: 14668438]
- 10.
- Tessier-Lavigne M, Goodman CS. The molecular biology of axon guidance. Science. 1996;274:1123–33. [PubMed: 8895455]
- 11.
- Polleux F, Morrow T, Ghosh A. Semaphorin 3A is a chemoattractant for cortical apical dendrites. Nature. 2000;404:567–73. [PubMed: 10766232]
- 12.
- Kim S, Chiba A. Dendritic guidance. Trends Neurosci. 2004;27:194–202. [PubMed: 15046878]
- 13.
- Song HJ, Poo MM. Signal transduction underlying growth cone guidance by diffusible factors. Curr Opin Neurobiol. 1999;9:355–63. [PubMed: 10395576]
- 14.
- Tessier-Lavigne M. Axon guidance by molecular gradients. Curr Opin Neurobiol. 1992;2:60–65. [PubMed: 1638137]
- 15.
- Song H, Poo M. The cell biology of neuronal navigation. Nat Cell Biol. 2001;3:E81–88. [PubMed: 11231595]
- 16.
- Gundersen RW, Barrett JN. Characterization of the turning response of dorsal root neurites toward nerve growth factor. J Cell Biol. 1980;87:546–54. [PMC free article: PMC2110791] [PubMed: 6257725]
- 17.
- Ming GL, et al. Adaptation in the chemotactic guidance of nerve growth cones. Nature. 2002;417:411–18. [PubMed: 11986620]
- 18.
- Campbell DS, Holt CE. Chemotropic responses of retinal growth cones mediated by rapid local protein synthesis and degradation. Neuron. 2001;32:1013–26. [PubMed: 11754834]
- 19.
- Wu KY, et al. Local translation of RhoA regulates growth cone collapse. Nature. 2005;436:1020–24. [PMC free article: PMC1317112] [PubMed: 16107849]
- 20.
- Campbell DS, Holt CE. Apoptotic pathway and MAPKs differentially regulate chemotropic responses of retinal growth cones. Neuron. 2003;37:939–52. [PubMed: 12670423]
- 21.
- Jellies J, Loer CM, Kristan WB Jr. Morphological changes in leech Retzius neurons after target contact during embryogenesis. J Neurosci. 1987;7:2618–29. [PMC free article: PMC6569139] [PubMed: 3625266]
- 22.
- Zheng JQ, Zheng Z, Poo M. Long-range signaling in growing neurons after local elevation of cyclic AMP-dependent activity. J Cell Biol. 1994;127:1693–701. [PMC free article: PMC2120268] [PubMed: 7798321]
- 23.
- Davenport RW, Thies E, Cohen ML. Neuronal growth cone collapse triggers lateral extensions along trailing axons. Nat Neurosci. 1999;2:254–59. [PubMed: 10195218]
- 24.
- Henley J, Poo MM. Guiding neuronal growth cones using Ca2+ signals. Trends Cell Biol. 2004;14:320–30. [PMC free article: PMC3115711] [PubMed: 15183189]
- 25.
- Kater SB, Mills LR. Regulation of growth cone behavior by calcium. J Neurosci. 1991;11:891–99. [PMC free article: PMC6575390] [PubMed: 2010811]
- 26.
- Kater SB, Davenport RW, Guthrie PB. Filopodia as detectors of environmental cues: signal integration through changes in growth cone calcium levels. Prog Brain Res. 1994;102:49–60. [PubMed: 7800832]
- 27.
- Gomez TM, Robles E, Poo M, Spitzer NC. Filopodial calcium transients promote substrate-dependent growth cone turning. Science. 2001;291:1983–87. [PubMed: 11239161]
- 28.
- Lohof AM, Quillan M, Dan Y, Poo MM. Asymmetric modulation of cytosolic cAMP activity induces growth cone turning. J Neurosci. 1992;12:1253–61. [PMC free article: PMC6575801] [PubMed: 1372932]
- 29.
- Hong K, Nishiyama M, Henley J, Tessier-Lavigne M, Poo M. Calcium signalling in the guidance of nerve growth by netrin-1. Nature. 2000;403:93–98. [PubMed: 10638760]
- 30.
- Ming G, Henley J, Tessier-Lavigne M, Song H, Poo M. Electrical activity modulates growth cone guidance by diffusible factors. Neuron. 2001;29:441–52. [PubMed: 11239434]
- 31.
- Xu HT, et al. Calcium signaling in chemorepellant slit2-dependent regulation of neuronal migration. Proc Natl Acad Sci USA. 2004;101:4296–301. [PMC free article: PMC384735] [PubMed: 15020772]
- 32.
- Jin M, et al. Ca2+-dependent regulation of rho GTPases triggers turning of nerve growth cones. J Neurosci. 2005;25:2338–47. [PMC free article: PMC6726106] [PubMed: 15745960]
- 33.
- Xiang Y, et al. Nerve growth cone guidance mediated by G-protein-coupled receptors. Nat Neurosci. 2002;5:843–48. [PubMed: 12161754]
- 34.
- Zheng JQ. Turning of nerve growth cones induced by localized increases in intracellular calcium ions. Nature. 2000;403:89–93. [PubMed: 10638759]
- 35.
- Clapham DE, Runnels LW, Strübing C. The TRP ion channel family. Nat Rev Neurosci. 2001;2:387–96. [PubMed: 11389472]
- 36.
- Ming GL, et al. cAMP-dependent growth cone guidance by netrin-1. Neuron. 1997;19:1225–35. [PubMed: 9427246]
- 37.
- Nishiyama M, et al. Cyclic AMP/GMP-dependent modulation of Ca2+ channels sets the polarity of nerve growth-cone turning. Nature. 2003;423:990–95. [PubMed: 12827203]
- 38.
- McFarlane S, Pollock NS. A role for voltage-gated potassium channels in the outgrowth of retinal axons in the developing visual system. J Neurosci. 2000;20:1020–29. [PMC free article: PMC6774185] [PubMed: 10648707]
- 39.
- Pollock NS, et al. Voltage-gated potassium channels regulate the response of retinal growth cones to axon extension and guidance cues. Eur J Neurosci. 2005;22:569–78. [PubMed: 16101738]
- 40.
- Li Y, et al. Essential role of TRPC channels in the guidance of nerve growth cones by brain-derived neurotrophic factor. Nature. 2005;434:894–98. [PubMed: 15758952]
- 41.
- Wang GX, Poo MM. Requirement of TRPC channels in netrin-1-induced chemotropic turning of nerve growth cones. Nature. 2005;434:898–904. [PubMed: 15758951]
- 42.
- Shim S, et al. XTRPC1-dependent chemotropic guidance of neuronal growth cones. Nat Neurosci. 2005;8:730–35. [PMC free article: PMC4005724] [PubMed: 15880110]
- 43.
- Huang EJ, Reichardt LF. Trk receptors: roles in neuronal signal transduction. Annu Rev Biochem. 2003;72:609–42. [PubMed: 12676795]
- 44.
- Ordaz B, et al. Calmodulin and calcium interplay in the modulation of TRPC5 channel activity. Identification of a novel C-terminal domain for calcium/calmodulin-mediated facilitation. J Biol Chem. 2005;280:30788–96. [PubMed: 15987684]
- 45.
- Montell C. PLC fills a GAP in G-protein-coupled signalling. Nat Cell Biol. 2000;2:E82–83. [PubMed: 10806493]
- 46.
- Rebecchi MJ, Pentyala SN. Structure, function, and control of phosphoinositide-specific phospholipase C. Physiol Rev. 2000;80:1291–335. [PubMed: 11015615]
- 47.
- Li HS, Xu XZ, Montell C. Activation of a TRPC3-dependent cation current through the neurotrophin BDNF. Neuron. 1999;24:261–73. [PubMed: 10677043]
- 48.
- Trebak M, Vazquez G, Bird GS, Putney JW Jr. The TRPC3/6/7 subfamily of cation channels. Cell Calcium. 2003;33:451–61. [PubMed: 12765690]
- 49.
- Hofmann T, Schaefer M, Schultz G, Gudermann T. Subunit composition of mammalian transient receptor potential channels in living cells. Proc Natl Acad Sci USA. 2002;99:7461–66. [PMC free article: PMC124253] [PubMed: 12032305]
- 50.
- Goel M, Sinkins WG, Schilling WP. Selective association of TRPC channel subunits in rat brain synaptosomes. J Biol Chem. 2002;277:48303–10. [PubMed: 12377790]
- 51.
- Yamada H, et al. Spontaneous single-channel activity of neuronal TRP5 channel recombinantly expressed in HEK293 cells. Neurosci Lett. 2000;285:111–14. [PubMed: 10793239]
- 52.
- Jung S, et al. Lanthanides potentiate TRPC5 currents by an action at extracellular sites close to the pore mouth. J Biol Chem. 2003;278:3562–71. [PubMed: 12456670]
- 53.
- Zitt C, et al. Expression of TRPC3 in Chinese hamster ovary cells results in calcium-activated cation currents not related to store depletion. J Cell Biol. 1997;138:1333–41. [PMC free article: PMC2132548] [PubMed: 9298988]
- 54.
- Guan KL, Rao Y. Signalling mechanisms mediating neuronal responses to guidance cues. Nat Rev Neurosci. 2003;4:941–56. [PubMed: 14682358]
- 55.
- Komuro H, Rakic P. Intracellular Ca2+ fluctuations modulate the rate of neuronal migration. Neuron. 1996;17:275–85. [PubMed: 8780651]
- 56.
- Kumada T, Komuro H. Completion of neuronal migration regulated by loss of Ca(2+) transients. Proc Natl Acad Sci USA. 2004;101:8479–84. [PMC free article: PMC420419] [PubMed: 15150416]
- 57.
- Komuro H, Rakic P. Orchestration of neuronal migration by activity of ion channels, neurotransmitter receptors, and intracellular Ca2+ fluctuations. J Neurobiol. 1998;37:110–30. [PubMed: 9777736]
- 58.
- Komuro H, Kumada T. Ca2+ transients control CNS neuronal migration. Cell Calcium. 2005;37:387–93. [PubMed: 15820385]
- 59.
- Rakic P, Komuro H. The role of receptor/channel activity in neuronal cell migration. J Neurobiol. 1995;26:299–315. [PubMed: 7775964]
- 60.
- Komuro H, Rakic P. Dynamics of granule cell migration: a confocal microscopic study in acute cerebellar slice preparations. J Neurosci. 1995;15:1110–20. [PMC free article: PMC6577849] [PubMed: 7869087]
- 61.
- Komuro H, Rakic P. Distinct modes of neuronal migration in different domains of developing cerebellar cortex. J Neurosci. 1998;18:1478–90. [PMC free article: PMC6792738] [PubMed: 9454856]
- 62.
- Sigurdson WJ, Morris CE. Stretch-activated ion channels in growth cones of snail neurons. J Neurosci. 1989;9:2801–8. [PMC free article: PMC6569684] [PubMed: 2475592]
- 63.
- Imai K, Tatsumi H, Katayama Y. Mechanosensitive chloride channels on the growth cones of cultured rat dorsal root ganglion neurons. Neuroscience. 2000;97:347–55. [PubMed: 10799766]
- 64.
- Bray D. Axonal growth in response to experimentally applied mechanical tension. Dev Biol. 1984;102:379–89. [PubMed: 6706005]
- 65.
- Chada S, Lamoureux P, Buxbaum RE, Heidemann SR. Cytomechanics of neurite outgrowth from chick brain neurons. J Cell Sci. 1997;110(Pt. 10):1179–86. [PubMed: 9191042]
- 66.
- Zheng J, et al. Tensile regulation of axonal elongation and initiation. J Neurosci. 1991;11:1117–25. [PMC free article: PMC6575379] [PubMed: 2010807]
- 67.
- Zheng J, Buxbaum RE, Heidemann SR. Investigation of microtubule assembly and organization accompanying tension-induced neurite initiation. J Cell Sci. 1993;104(Pt. 4):1239–50. [PubMed: 8314903]
- 68.
- Lamoureux P, Ruthel G, Buxbaum RE, Heidemann SR. Mechanical tension can specify axonal fate in hippocampal neurons. J Cell Biol. 2002;159:499–508. [PMC free article: PMC2173080] [PubMed: 12417580]
- 69.
- Munevar S, Wang YL, Dembo M. Regulation of mechanical interactions between fibroblasts and the substratum by stretch-activated Ca2+ entry. J Cell Sci. 2004;117:85–92. [PubMed: 14627625]
- 70.
- Calabrese B, Tabarean IV, Juranka P, Morris CE. Mechanosensitivity of N-type calcium channel currents. Biophys J. 2002;83:2560–74. [PMC free article: PMC1302342] [PubMed: 12414690]
- 71.
- Komuro H, Rakic P. Selective role of N-type calcium channels in neuronal migration. Science. 1992;257:806–9. [PubMed: 1323145]
- 72.
- Tousova K, Vyklicky L, Susankova K, Benedikt J, Vlachova V. Gadolinium activates and sensitizes the vanilloid receptor TRPV1 through the external protonation sites. Mol Cell Neurosci. 2005;30:207–17. [PubMed: 16099171]
- 73.
- Lin SY, Corey DP. TRP channels in mechanosensation. Curr Opin Neurobiol. 2005;15:350–57. [PubMed: 15922584]
- 74.
- Komuro H, Yacubova E, Rakic P. Mode and tempo of tangential cell migration in the cerebellar external granular layer. J Neurosci. 2001;21:527–40. [PMC free article: PMC6763794] [PubMed: 11160432]
- 75.
- Guatteo E, et al. Temperature sensitivity of dopaminergic neurons of the substantia nigra pars compacta: involvement of TRP channels. J Neurophysiol. 2005 [PubMed: 16014800]
- TRP Channels and Axon Pathfinding - TRP Ion Channel Function in Sensory Transduc...TRP Channels and Axon Pathfinding - TRP Ion Channel Function in Sensory Transduction and Cellular Signaling Cascades
Your browsing activity is empty.
Activity recording is turned off.
See more...