NCBI Bookshelf. A service of the National Library of Medicine, National Institutes of Health.
Liedtke WB, Heller S, editors. TRP Ion Channel Function in Sensory Transduction and Cellular Signaling Cascades. Boca Raton (FL): CRC Press/Taylor & Francis; 2007.
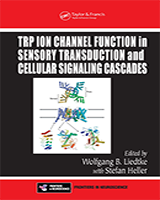
TRP Ion Channel Function in Sensory Transduction and Cellular Signaling Cascades.
Show detailsINTRODUCTION
TRPV4, the fourth member of the vanilloid subfamily of TRP channels, is a calcium-permeable cation channel that is detectable in both sensory and nonsensory cells. It has been shown to be widely expressed, including in kidneys, lungs, hearts, brains, endothelial cells, and dorsal root and trigeminal sensory ganglia. The channel is characterized by multimodal activation properties that implicate it in a broad range of functions from osmoregulation to thermosensing. Although TRPV4 was originally identified as an osmotically activated channel [1–3], recent evidence demonstrates that the channel can be activated by diverse stimuli including hypoosmotic swelling [1–3], shear stress [4], nonnoxious temperatures [5,6], acidity [7], phorbol esters (both protein kinase C–activating and nonactivating phorbol esters) [4,8,9], and downstream metabolites of arachidonic acid (epoxyeicosatrienoic acids) [10,11]. The mechanism(s) underlying TRPV4 activation by these differing modalities remain(s) poorly understood. However, new evidence points to some common pathways of activation by these diverse stimuli that may underlie the apparent broad range of functions associated with the channel.
TRPV4 STRUCTURE
The 871 amino acids (aa) that make up the most common isoform of TRPV4 are predicted to form the individual subunits of the functional homotetrameric ion channel. Amino and carboxyl termini of TRPV4 are localized in the cytoplasm and flank the core region consisting of six transmembrane-spanning domains (TM1–6) and a pore loop between TM5 and TM6 [1–3].
Determination of the structure of the bacterial P-loop KcsA potassium channel [12,13], as well as other recently determined structures for KirBac1.1 [14] and KirBac3.1 [15], has provided new insights into the general structure of P-loop channels, prompting speculation as to the potential structure of TRPV channels [16]. P-loop channels typically form as tetramers, consistent with TRP channel structure, with a four-fold symmetry around the central pore region [17,18].
Amino Terminus
More than half of the TRPV4 protein is formed by the intracellularly located amino terminus that most prominently harbors three ankyrin repeat domains (ARD1–3) within an ankyrin repeat region from aa235 to aa367, a cluster of four protein kinase C (PKC)–phosphorylation sites and a cAMP-dependent–phosphorylation site upstream of the ankyrin repeat region, and a cluster of two PKC sites within and downstream of ARD3 (Figure 8.1A). A functionally identified Src family dependent tyrosine phosphorylation site has been described at aa253 within ARD1. Other potentially functionally important motifs are an N-myristoylation site at aa24–29, a bipartite nuclear targeting sequence that overlaps with part of ARD3, and a PKC phosphorylation site.
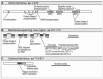
FIGURE 8.1
Schematic overview of TRPV4’s predicted structural and functional components. Shown are schematic representations of TRPV4’s amino terminus (A), its central region with the membrane-spanning domains and the pore loop (B), and the channel’s (more...)
It has been hypothesized that increased PKC activity in response to mechanical stress could be a factor in TRPV4 activation. Activation of PKC with phorbol esters leads to opening of TRPV4 and increase of intracellular Ca2+ concentration when the experiment is conducted at 37°C [4,9]. At room temperature, PKC-activating phorbol esters have no or very little effect on TRPV4 gating. The sites of PKC action on TRPV4 are unknown. Candidates for direct phosphorylation are the six PKC phosphorylation sites in the amino terminus and one motif just downstream of TM2 (Figure 8.1A–B). Another motif that has been implicated in regulating TRPV4 is a single Src family tyrosine phosphorylation site within ARD1 that is phosphorylated in response to hypotonic stress [19]. A Y253F point mutant exhibited nearly abolished hypotonicity responses [19], a result that recently has been challenged [20,21].
Membrane-Spanning Core Region and Pore Loop
The 242-aa-long central domain of TRPV4 consists of TM1–6, which feature, between TM5 and TM6, a short hydrophobic stretch that is the putative pore region or pore loop (PL, Figure 8.1B). TRPV4 does display only a few predicted short extracellular domains, 25 aa between TM1 and TM2, 4 aa between TM3 and TM4, and 34 aa and 12 aa upstream and downstream of the PL. The channel appears to be posttranslationally modified by glycosylation [22], and a bona fide Asn glycosylation site within the extracellular stretch between TM5 and the PL has been shown to be glycosylated in heterologously expressed TRPV4 [23]. A PKC phosphorylation site downstream of TM2 is potentially involved in the above-mentioned PKC regulation of TRPV4 activation.
By analogy to the bacterial KcsA K+ channel [12,13], the PL is probably composed of a short highly hydrophobic pore helix immediately followed by an ion selectivity segment, the channel selectivity filter, that has been postulated to encompass aa672–682 [16]. Several individual aas affect the selectivity and permeability; when mutated (notably aspartate residues D672 and D682, and, somewhat surprisingly, M680), they markedly impair calcium permeation, results are consistent with the purported selectivity filter of TRPV4 (see below).
Carboxyl Terminus
TRPV4’s carboxyl terminal tail appears to be the docking site for at least two interacting proteins. One of these sites has been narrowed down to a region between aa812 and aa831 and confers binding to calmodulin [24]. Mutations within this region resulted in a loss of Ca2+-dependent calmodulin binding and a loss of Ca2+-dependent potentiation of TRPV4 currents. This observed effect adds to the complex Ca2+ dependence of TRPV4 activity that also involves inhibitory effects [1,25].
Immediately upstream of the calmodulin-binding region, an interaction site with microfilament-associated protein 7 (MAP7) has been proposed. Coexpression of TRPV4 with MAP7 in CHO cells apparently increases the amount of TRPV4 protein associated with the plasma membrane, which could be a method employed by cells to control the density of TRPV4 in the plasma membrane [26].
EMERGING MECHANISM OF CHANNEL ACTIVATION
TRPV4 is a multimodal channel regulated by a diverse array of stimuli, implicating multiple mechanisms of regulation and function. The potential cellular pathways underlying this regulation are outlined below.
Mechanosensitivity
The TRPV4 channel was initially cloned based on its sensitivity to hypoosmotic cell swelling, implicating the channel as a potential mechanosensitive channel [1–3] as mentioned. It was subsequently shown that application of fluid shear stress (or fluid flow) across the apical surface of TRPV4-expressing HEK293 epithelial cells and M-1 renal collecting duct cells activated the channel, confirming the mechanosensitive nature of activation [4,27]. The response to mechanical stimulation, however, was shown to be highly sensitive to temperature [1,4], implicating a temperature-induced augmentation or sensitization to mechanical stimuli. The mechanosensitive nature of TRPV4, or a TRPV4 complex, was most clearly demonstrated in a seminal study of C. elegans using osm-9 mutant worms lacking expression of a worm TRPV homologue, OSM-9, which underlies osmotic (hyperosmolarity) and mechanical (nose touch) avoidance behavior. Transgenically targeting expression of mammalian TRPV4 to the ASH amphid sensory neuron in the mutants (a normal site of OSM-9 expression) rescued the osmotic and mechanical avoidance responses, providing compelling evidence that in the in vivo setting, TRPV4 functions as an osmosensitive and mechanosensitive channel or sensory complex [28].
The mechanism by which the TRPV4 channel is activated by mechanical stresses is currently not fully understood. Two, not mutually exclusive, principal mechanisms have been proposed: direct mechanical activation and indirect activation through other transduction pathways. Cell swelling can lead to activation of both the phospholipase C (PLC)/diacylglycerol (DAG) transduction pathway [29,30] and the phospholipase A2 (PLA2)/arachidonic acid (AA) transduction pathway [31–33], two pathways that can modulate, or regulate, TRPV4 activation (see below). However, Nilius and coworkers demonstrated, in a detailed series of studies, that swelling-induced production of AA and its downstream epoxyeicosatrienoic acid (EET) metabolites (5,6-EET and 8,9-EET) may be responsible, at least in part, for hypotonic-induced activation of TRPV4 [10,11,21]. Inhibition of PLA2 or cytochrome P450 epoxygenase activity reduced the swelling-induced activation of TRPV4, while application of the EET metabolites or inhibition of EET hydrolysis enhanced both the swelling and AA-induced activation of TRPV4. TRPV4 knockout mice demonstrated a reduced swelling and AA-induced activation of calcium entry in mouse aortic endothelial cells [10]. Hence, the PLA2–cytochrome P450 pathway may reflect a dominant pathway and mechanism by which mechanical stress may activate the channel. However, direct application of membrane stretch per se on channel activation, such as recently demonstrated for TRPC1 [34], without input from arachidonic acid and its metabolites, or from DAG and its metabolites, has not been systematically tested and remains to be determined, particularly at physiologically relevant temperatures.
The mechanosensory functions of TRPV4 in mammals are slowly being elucidated as described in more detail previously [35,36] and in other chapters of this volume. As noted, TRPV4 has been shown to be activated by cell swelling [1–3], implicating a potential role in cell volume regulation [37], and in sensing shear stress, where the channel may play a key sensory role in flow-sensitive tissues such as vascular endothelial cells and renal tubular epithelial cells [4,6,27]. The channel has been strongly implicated as a mechanosensor in cilia of oviductal epithelial cells, showing an increased channel activity and accompanying Ca2+ influx, following an increase in fluid viscosity (i.e., increased mechanical load) [38]. In addition, hypotonicity-induced nociception has also been described as TRPV4-mediated [39]. Further, studies employing TRPV4-deficient knockout mice have implicated TRPV4 in specific functions: in sensing plasma osmolarity by the sensory circumventricular organs of the hypothalamus, which, in turn, regulate secretion of antidiuretic hormone and control of extracellular fluid osmolarity; [40,41] in sensing tail pressure by dorsal root ganglia sensory neurons, implicating TRPV4 as a high threshold mechanoreceptor; [7] and in sensing hypotonic cell volume in mouse aortic endothelial cells [10]. Other functions of TRPV4 are continuing to emerge, and many more are likely to be forthcoming [35,36].
Thermosensitivity
The survival of animals, especially warm-blooded animals, relies on the animals’ abilities to sense and respond to changes in temperature. TRP channels may be central components of this sensing ability. The TRPV channel members, TRPV1–4, have been shown to be activated by warm to noxious temperatures and have been implicated in temperature sensing by the body. The TRPV channels display defined temperature thresholds for activation: TRPV1, 42°C; [42,43] TRPV2, 52°C; [44] TRPV3, 31°C; [45–47] and TRPV4, near 27–35°C [5,6]. The channels have recently been dubbed “thermoTRPs” because of this feature [48]. These investigators demonstrated that the mechanism of temperature dependency may relate to a temperature-dependent shift of the channels’ voltage dependency (normally not a notable feature of TRP channels) to more physiologically relevant voltage ranges. That is, elevating the temperature for warm/hot TRPVs provides a left shift in the voltage sensitivity, making the voltage dependency apparent at more “physiological” voltages, thereby activating the channel. (Cool/cold-activated TRPs displayed a complementary right shift in the voltage sensitivity with cooling.) TRPV4, however, does not specifically fit this model, as it could not be demonstrated to be temperature sensitive when studied in patch-clamp studies where the membrane was detached from the cell [48]. Other cellular factors, yet to be identified, may be critical for the TRPV4 channel to display temperature-induced activation.
The physiological function of TRPV4 in thermosensation is still emerging [48,49]. Besides functioning as a general sensor of altered cell/tissue temperature, TRPV4 expression in skin keratinocytes has been implicated as a sensor of “warm” temperatures [50]. TRPV4 knockout studies clearly implicate TRPV4 in selecting “preferred” footpad temperatures and as being essential in thermal hyperalgesia [51].
Diacylglycerol and Phorbol Esters
The first ligand discovered to activate TRPV4 was the synthetic phorbol ester, 4α-phorbol 12,13-didecanoate (4α-PDD) [8]. It was observed that 4α-PDD could potently activate TRPV4, even at relatively modest concentrations (<1 μM), and it appeared to be relatively specific for TRPV4 because it does not appear to activate other TRPV family members. Since 4α-PDD is a non-protein kinase C–activating phorphol ester that is not metabolizable, it may activate TRPV4 by directly binding to the channel or an associated subunit. However, recent studies by Xu and coworkers [9] have demonstrated that other inactive 4α isomers can activate the channel with a potency that appears to be related to their chemical hydrophobicity. This may indicate a potential effect of the phorbol isomers on the lipid environment in modulating, or activating, the channel. The physiological relevance of such a lipid-modulated mechanism of activation may have broad implications on the function and mechanisms of TRPV4 gating as noted for other mechanogated channels [52,53].
The PKC-activating phorbol esters were originally considered only to activate TRPV4 weakly based on studies done at room temperature [8]. It was subsequently demonstrated that elevation of temperature to the physiological range resulted in a potent augmentation, or sensitization, of the channel to phorbol esters and mechanical stimuli [4]. At 37°C, PMA in the submicromolar range activated TRPV4 in a PKC-dependent manner, approaching the potency of 4α-PDD [4,9]. Other PKC-activating phorbol esters (phorbol 12,13-didecanoate, PDD; phorbol 12,13-dibutyrate, PDBu) [9] similarly activated the channel in a PKC-dependent manner, implicating PKC as an important modulator of TRPV4 activity. These studies raise the exciting possibility that signaling pathways underlying induction of PKC, such as G-protein-coupled receptors or tyrosine kinase receptors linked to activation of phospholipase C, may reflect emerging, as yet undiscovered, pathways for TRPV4 activation.
Arachidonic Acid and Epoxygenase Metabolites
The discovery that arachidonic acid (AA) and its downstream metabolites may activate TRPV4 provided the first evidence for endogenous agonists of the channel. It was initially shown by Nilius and coworkers [11] that the endocannabinoid ananda-mide (AEA) and its lipoxygenase (fatty acid amidohydrolase) metabolite, AA, could activate TRPV4. A nonmetabolizable analogue of AEA (e.g., methanandamide) was without effect, while an inhibitor of fatty acid amidohydrolase, phenylmethylsulfonyl fluoride, abolished the AEA-induced activation of TRPV4. It was subsequently shown that generating EET metabolites of AA via the cytochrome P450 epoxygenase pathway, notably 5,6-EET and 8,9-EET, could activate TRPV4 [10,21]. Other studies have shown that the 11,12-EET may similarly activate TRPV4 [54]. Furthermore, activation by AA metabolites appears to be distinct from the 4α-PDD pathway of activation because mutation of a tyrosine residue near the third transmembrane domain (Y555, Figure 8.1B) to an alanine impairs activation by 4α-PDD, but not by AA or cell swelling, implicating a potential role for tyrosine phosphorylation in the activation pathway for 4α-PDD [21]. Recent studies in mouse vascular endothelial cells, which express endogenous TRPV4, further demonstrate that inhibiting EET hydrolysis also leads to an enhanced response of AA or EET analogues, while in cells isolated from animals lacking TRPV4 (TRPV4−/−), all TRPV4 agonists are without effect [10]. Hence, accumulating evidence points to the AA pathway and the epoxygenase metabolites as playing key roles in regulating TRPV4 activity in the endogenous setting.
MOLECULAR INSIGHTS INTO CHANNEL SELECTIVITY AND GATING
The selectivity filter and gating mechanism of TRPV4 have not been determined. Insights into these mechanisms are beginning to emerge from recent studies of TRPV4 and other P-loop channels.
The Molecular Basis of Selectivity
The molecular basis of the TRPV4 channel selectivity filter has not been fully elucidated, although charged residues in the purported filter segment, aa672–682, have been implicated. In particular, two aspartate residues (D672 and D682) appear to cooperatively affect the pore’s Ca2+ permeability and channel rectification [55]. Nevertheless, these two aas are not strictly linked as mutations to neutralize D672 alone does not have any strong effects, and neutralization of D682 but not D672 almost abolishes the blocking effect of ruthenium red [20,55].
Nilius and colleagues [16,20] have hypothesized that the structural design of the TRPV4 pore is comparable to that of the bacterial KcsA K+ channel [12,13]. Based on this assessment, the PL is characterized by a short pore helix followed by the selectivity filter (Figure 8.1B). This notion was spurred by the weak but noticeable similarity of the TIGMGD stretch of aa677–aa682 (Figure 8.1B) within the PL with the TXGYGD selectivity filter residues of the K+ channel signature sequence [12,13]. The aforementioned mutations of D672 and D682, as well as M680, which impair Ca2+ permeation and reduce whole cell current amplitude, circumstantially support this hypothesis and clearly demonstrate involvement of this segment of the PL in ion selectivity and permeability [20,55].
Emerging Paradigms of Gating
The conformational changes in channel structure that lead to functional transitions from closed to open conducting states (i.e. “gating”) are not well understood for most channels. Again, based on structural analysis of bacterial K+ channels [12–15], potential TRPV4 gating mechanisms can be suggested [16]. The tetrameric subunit arrangement provides for fourfold symmetry around the central pore region [17,18], where an inner transmembrane helical segment from each of the four subunits each arranges concentrically to form the channel barrel or pore. The transmembrane helices are tilted inward to form a cone shape of the barrel with narrowing toward the cytoplasmic face. This narrowing of the transmembrane segments toward the cytoplasmic face forms the main activation gate [17,18,56]. Hinging movements of these segments can widen the pore, thereby opening the gate, or can narrow the pore, thereby closing the gate. The overall structure of the TRPV channels, including TRPV4, would be consistent with such a gating structure, as speculated by others [16], although this model for TRPV4 gating has not been explicitly evaluated.
Other components of the structure of P-loop channels, notably the pore helix, may also play a role in the gating process. Rotation or movement of the pore helix can modulate gating, or perhaps act as a secondary gate, in some P-loop channels [57,58]. Recent studies of TRPV5 gating, using the cysteine-accessibility method, have shown that inhibiting the channel by internal hydrogen is associated with a clockwise rotation of the pore helix along its long axis [59]. It is implicit in these findings that such rotational movements of the pore helix may be critical for gating other TRPV channels, such as TRPV4. Therefore, it is reasonable to conclude that, at a minimum, both hinging movements of the helices forming the activation gate at the cytoplasm face and rotation of the pore helices of the pore loop regions are components of TRPV channel gates. For multimodal channels, such as TRPV4, it may be that other structural components to gating may also be at play. Hence, future studies should provide exciting new insights into the molecular mechanisms of gating of TRPV4 and other TRP channels.
SUMMARY
In the five years since TRPV4 was first described, substantial progress has been made toward understanding this channel’s roles in cellular and physiologic processes. These studies revealed that TRPV4 is a rather complex ion channel, capable of integrating multiple physical stimuli such as mechanic stress and temperature. In addition, the channel’s gating is considerably modulated by phosphorylation, external and internal Ca2+ concentration, PLC activity, and AA metabolites, which are potentially generated via the PLA2 pathway. The strong activating capacity of certain synthetic non-PKC-activating phorbol esters adds lipid modulation as an intriguing additional aspect of TRPV4 activation. Future structure and function studies, not only of TRPV4 but also of other TRP channels, will shed more light on the physiological purpose of the apparently complex gating and modulation of TRPV4.
REFERENCES
- 1.
- Liedtke W, Choe Y, Marti-Renom MA, Bell AM, Denis CS, Sali A, Hudspeth AJ, Friedman JM, Heller S. Vanilloid receptor–related osmotically activated channel (VR-OAC), a candidate vertebrate osmoreceptor. Cell. 2000;103(3):525–35. [PMC free article: PMC2211528] [PubMed: 11081638]
- 2.
- Strotmann R, Harteneck C, Nunnenmacher K, Schultz G, Plant TD. OTRPC4, a nonselective cation channel that confers sensitivity to extracellular osmolarity. Nat Cell Biol. 2000;2(10):695–702. [PubMed: 11025659]
- 3.
- Wissenbach U, Bodding M, Freichel M, Flockerzi V. Trp12, a novel Trp-related protein from kidney. FEBS Lett. 2000;485(2-3):127–34. [PubMed: 11094154]
- 4.
- Gao X, Wu L, O’Neil RG. Temperature-modulated diversity of TRPV4 channel gating: activation by physical stresses and phorbol ester derivatives through protein kinase C–dependent and –independent pathways. J Biol Chem. 2003;278(29):27129–37. [PubMed: 12738791]
- 5.
- Guler AD, Lee H, Iida T, Shimizu I, Tominaga M, Caterina M. Heat-evoked activation of the ion channel, TRPV4. J Neurosci. 2002;22(15):6408–14. [PMC free article: PMC6758176] [PubMed: 12151520]
- 6.
- Watanabe H, Vriens J, Suh SH, Benham CD, Droogmans G, Nilius B. Heat-evoked activation of TRPV4 channels in a HEK293 cell expression system and in native mouse aorta endothelial cells. J Biol Chem. 2002;277(49):47044–51. [PubMed: 12354759]
- 7.
- Suzuki M, Mizuno A, Kodaira K, Imai M. Impaired pressure sensation in mice lacking TRPV4. J Biol Chem. 2003;278(25):22664–68. [PubMed: 12692122]
- 8.
- Watanabe H, Davis JB, Smart D, Jerman JC, Smith GD, Hayes P, Vriens J, Cairns W, Wissenbach U, Prenen J, Flockerzi V, Droogmans G, Benham CD, Nilius B. Activation of TRPV4 channels (hVRL-2/mTRP12) by phorbol derivatives. J Biol Chem. 2002;277(16):13569–77. [PubMed: 11827975]
- 9.
- Xu F, Satoh E, Iijima T. Protein kinase C–mediated Ca2+ entry in HEK 293 cells transiently expressing human TRPV4. Br J Pharmacol. 2003;140(2):413–21. [PMC free article: PMC1574039] [PubMed: 12970074]
- 10.
- Vriens J, Owsianik G, Fisslthaler B, Suzuki M, Janssens A, Voets T, Morisseau C, Hammock BD, Fleming I, Busse R, Nilius B. Modulation of the Ca2 permeable cation channel TRPV4 by cytochrome P450 epoxygenases in vascular endothelium. Circ Res. 2005;97(9):908–15. [PubMed: 16179585]
- 11.
- Watanabe H, Vriens J, Prenen J, Droogmans G, Voets T, Nilius B. Anandamide and arachidonic acid use epoxyeicosatrienoic acids to activate TRPV4 channels. Nature. 2003;424(6947):434–38. [PubMed: 12879072]
- 12.
- Doyle DA, Morais Cabral J, Pfuetzner RA, Kuo A, Gulbis JM, Cohen SL, Chait BT, MacKinnon R. The structure of the potassium channel: molecular basis of K+ conduction and selectivity. Science. 1998;280(5360):69–77. [PubMed: 9525859]
- 13.
- Zhou Y, Morais-Cabral JH, Kaufman A, MacKinnon R. Chemistry of ion coordination and hydration revealed by a K+ channel-Fab complex at 2.0 A resolution. Nature. 2001;414(6859):43–48. [PubMed: 11689936]
- 14.
- Kuo A, Gulbis JM, Antcliff JF, Rahman T, Lowe ED, Zimmer J, Cuthbertson J, Ashcroft FM, Ezaki T, Doyle DA. Crystal structure of the potassium channel KirBac1.1 in the closed state. Science. 2003;300(5627):1922–26. [PubMed: 12738871]
- 15.
- Kuo A, Domene C, Johnson LN, Doyle DA, Venien-Bryan C. Two different conformational states of the KirBac3.1 potassium channel revealed by electron crystallography. Structure (Camb). 2005;13(10):1463–72. [PubMed: 16216578]
- 16.
- Owsianik G, Talavera K, Voets T, Nilius B. Permeation and selectivity of TRP channels. Annu Rev Physiol. 2005 [PubMed: 16460288]
- 17.
- Doyle DA. Structural changes during ion channel gating. Trends Neurosci. 2004;27(6):298–302. [PubMed: 15165732]
- 18.
- MacKinnon R. Potassium channels. FEBS Lett. 2003;555(1):62–65. [PubMed: 14630320]
- 19.
- Xu H, Zhao H, Tian W, Yoshida K, Roullet JB, Cohen DM. Regulation of a transient receptor potential (TRP) channel by tyrosine phosphorylation. SRC family kinase-dependent tyrosine phosphorylation of TRPV4 on TYR-253 mediates its response to hypotonic stress. J Biol Chem. 2003;278(13):11520–27. [PubMed: 12538589]
- 20.
- Nilius B, Vriens J, Prenen J, Droogmans G, Voets T. TRPV4 calcium entry channel: a paradigm for gating diversity. Am J Physiol Cell Physiol. 2004;286(2):C195–205. [PubMed: 14707014]
- 21.
- Vriens J, Watanabe H, Janssens A, Droogmans G, Voets T, Nilius B. Cell swelling, heat, and chemical agonists use distinct pathways for the activation of the cation channel TRPV4. Proc Natl Acad Sci USA. 2004;101(1):396–401. [PMC free article: PMC314196] [PubMed: 14691263]
- 22.
- Arniges M, Fernandez-Fernandez JM, Albrecht N, Schaefer M, Valverde MA. Human TRPV4 channel splice variants revealed a key role of ankyrin domains in multimerization and trafficking. J Biol Chem. 2006;281:8996. [PubMed: 16293632]
- 23.
- Xu H, Fu Y, Tian W, Cohen DM. Glycosylation of the osmoresponsive transient receptor potential channel TRPV4 on Asn-651 influences membrane trafficking. Am J Physiol Renal Physiol. 2006;290:F1103–1109. [PubMed: 16368742]
- 24.
- Strotmann R, Schultz G, Plant TD. Ca2+-dependent potentiation of the non-selective cation channel TRPV4 is mediated by a C-terminal calmodulin-binding site. J Biol Chem. 2003;278(29):26541–49. [PubMed: 12724311]
- 25.
- Watanabe H, Vriens J, Janssens A, Wondergem R, Droogmans G, Nilius B. Modulation of TRPV4 gating by intra- and extracellular Ca2+ Cell Calcium. 2003;33(5-6):489–95. [PubMed: 12765694]
- 26.
- Suzuki M, Hirao A, Mizuno A. Microtubule-associated [corrected] protein 7 increases the membrane expression of transient receptor potential vanilloid 4 (TRPV4). J Biol Chem. 2003;278(51):51448–53. [PubMed: 14517216]
- 27.
- O’Neil RG, Wu L, Gao X. Mechanosensitive nature of the TRPV4 channel in renal epithelia revealed by siRNA gene silencing. Faseb J. 2005;19:A1163.
- 28.
- Liedtke W, Tobin DM, Bargmann CI, Friedman JM. Mammalian TRPV4 (VR-OAC) directs behavioral responses to osmotic and mechanical stimuli in Caenorhabditis elegans. Proc Natl Acad Sci USA. 2003;100(Suppl 2):14531–36. [PMC free article: PMC304114] [PubMed: 14581619]
- 29.
- O’Neil RG, Leng L. Osmo-mechanically sensitive phosphatidylinositol signaling regulates a Ca2+ influx channel in renal epithelial cells. Am J Physiol. 1997;273(1 Pt. 2):F120–28. [PubMed: 9249599]
- 30.
- Suzuki M, Kawahara K, Ogawa A, Morita T, Kawaguchi Y, Kurihara S, Sakai O. [Ca2+]i rises via G protein during regulatory volume decrease in rabbit proximal tubule cells. Am J Physiol. 1990;258(3 Pt. 2):F690–96. [PubMed: 2107759]
- 31.
- Basavappa S, Pedersen SF, Jorgensen NK, Ellory JC, Hoffmann EK. Swelling-induced arachidonic acid release via the 85-kDa cPLA2 in human neuroblastoma cells. J Neurophysiol. 1998;79(3):1441–49. [PubMed: 9497423]
- 32.
- Pedersen S, Lambert IH, Thoroed SM, Hoffmann EK. Hypotonic cell swelling induces translocation of the alpha isoform of cytosolic phospholipase A2 but not the gamma isoform in Ehrlich ascites tumor cells. Eur J Biochem. 2000;267(17):5531–39. [PubMed: 10951212]
- 33.
- Thoroed SM, Lauritzen L, Lambert IH, Hansen HS, Hoffmann EK. Cell swelling activates phospholipase A2 in Ehrlich ascites tumor cells. J Membr Biol. 1997;160(1):47–58. [PubMed: 9351891]
- 34.
- Maroto R, Raso A, Wood TG, Kurosky A, Martinac B, Hamill OP. TRPC1 forms the stretch-activated cation channel in vertebrate cells. Nat Cell Biol. 2005;7(2):179–85. [PubMed: 15665854]
- 35.
- Mutai H, Heller S. Vertebrate and invertebrate TRPV-like mechanoreceptors. Cell Calcium. 2003;33(5-6):471–78. [PubMed: 12765692]
- 36.
- O’Neil RG, Heller S. The mechanosensitive nature of TRPV channels. Pflügers Arch. 2005 [PubMed: 15909178]
- 37.
- Becker D, Blase C, Bereiter-Hahn J, Jendrach M. TRPV4 exhibits a functional role in cell-volume regulation. J Cell Sci. 2005;118(Pt. 11):2435–40. [PubMed: 15923656]
- 38.
- Andrade YN, Fernandes J, Vazquez E, Fernandez-Fernandez JM, Arniges M, Sanchez TM, Villalon M, Valverde MA. TRPV4 channel is involved in the coupling of fluid viscosity changes to epithelial ciliary activity. J Cell Biol. 2005;168(6):869–74. [PMC free article: PMC2171792] [PubMed: 15753126]
- 39.
- Alessandri-Haber N, Yeh JJ, Boyd AE, Parada CA, Chen X, Reichling DB, Levine JD. Hypotonicity induces TRPV4-mediated nociception in rat. Neuron. 2003;39(3):497–511. [PubMed: 12895423]
- 40.
- Liedtke W, Friedman JM. Abnormal osmotic regulation in trpv4−/− mice. Proc Natl Acad Sci USA. 2003;100(23):13698–703. [PMC free article: PMC263876] [PubMed: 14581612]
- 41.
- Mizuno A, Matsumoto N, Imai M, Suzuki M. Impaired osmotic sensation in mice lacking TRPV4. Am J Physiol Cell Physiol. 2003;285(1):C96–101. [PubMed: 12777254]
- 42.
- Caterina MJ, Schumacher MA, Tominaga M, Rosen TA, Levine JD, Julius D. The capsaicin receptor: a heat-activated ion channel in the pain pathway. Nature. 1997;389(6653):816–24. [PubMed: 9349813]
- 43.
- Jordt SE, Julius D. Molecular basis for species-specific sensitivity to ‘‘hot’’ chili peppers. Cell. 2002;108(3):421–30. [PubMed: 11853675]
- 44.
- Caterina MJ, Rosen TA, Tominaga M, Brake AJ, Julius D. A capsaicin-receptor homologue with a high threshold for noxious heat. Nature. 1999;398(6726):436–41. [PubMed: 10201375]
- 45.
- Peier AM, Reeve AJ, Andersson DA, Moqrich A, Earley TJ, Hergarden AC, Story GM, Colley S, Hogenesch JB, McIntyre P, Bevan S, Patapoutian A. A heat-sensitive TRP channel expressed in keratinocytes. Science. 2002;296(5575):2046–49. [PubMed: 12016205]
- 46.
- Smith GD, Gunthorpe MJ, Kelsell RE, Hayes PD, Reilly P, Facer P, Wright JE, Jerman JC, Walhin JP, Ooi L, Egerton J, Charles KJ, Smart D, Randall AD, Anand P, Davis JB. TRPV3 is a temperature-sensitive vanilloid receptor–like protein. Nature. 2002;418(6894):186–90. [PubMed: 12077606]
- 47.
- Xu H, Ramsey IS, Kotecha SA, Moran MM, Chong JA, Lawson D, Ge P, Lilly J, Silos-Santiago I, Xie Y, DiStefano PS, Curtis R, Clapham DE. TRPV3 is a calcium-permeable temperature-sensitive cation channel. Nature. 2002;418(6894):181–86. [PubMed: 12077604]
- 48.
- Voets T, Droogmans G, Wissenbach U, Janssens A, Flockerzi V, Nilius B. The principle of temperature-dependent gating in cold- and heat-sensitive TRP channels. Nature. 2004;430(7001):748–54. [PubMed: 15306801]
- 49.
- Tominaga M, Caterina MJ. Thermosensation and pain. J Neurobiol. 2004;61(1):3–12. [PubMed: 15362149]
- 50.
- Chung MK, Lee H, Caterina MJ. Warm temperatures activate TRPV4 in mouse 308 keratinocytes. J Biol Chem. 2003;278(34):32037–46. [PubMed: 12783886]
- 51.
- Todaka H, Taniguchi J, Satoh J, Mizuno A, Suzuki M. Warm temperature-sensitive transient receptor potential vanilloid 4 (TRPV4) plays an essential role in thermal hyperalgesia. J Biol Chem. 2004;279(34):35133–38. [PubMed: 15187078]
- 52.
- Anishkin A, Kung C. Microbial mechanosensation. Curr Opin Neurobiol. 2005;15(4):397–405. [PubMed: 16006117]
- 53.
- Kim D. Physiology and pharmacology of two-pore domain potassium channels. Curr Pharm Des. 2005;11(21):2717–36. [PubMed: 16101451]
- 54.
- Earley S, Heppner TJ, Nelson MT, Brayden JE. TRPV4 forms a novel Ca2+ signaling complex with ryanodine receptors and BKCa channels. Circ Res. 2005;97(12):1270–79. [PubMed: 16269659]
- 55.
- Voets T, Prenen J, Vriens J, Watanabe H, Janssens A, Wissenbach U, Bodding M, Droogmans G, Nilius B. Molecular determinants of permeation through the cation channel TRPV4. J Biol Chem. 2002;277(37):33704–10. [PubMed: 12093812]
- 56.
- Kung C, Blount P. Channels in microbes: so many holes to fill. Mol Microbiol. 2004;53(2):373–80. [PubMed: 15228520]
- 57.
- Liu J, Siegelbaum SA. Change of pore helix conformational state upon opening of cyclic nucleotide-gated channels. Neuron. 2000;28(3):899–909. [PubMed: 11163275]
- 58.
- Zhen XG, Xie C, Fitzmaurice A, Schoonover CE, Orenstein ET, Yang J. Functional architecture of the inner pore of a voltage-gated Ca2+ channel. J Gen Physiol. 2005;126(3):193–204. [PMC free article: PMC2266581] [PubMed: 16129770]
- 59.
- Yeh BI, Kim YK, Jabbar W, Huang CL. Conformational changes of pore helix coupled to gating of TRPV5 by protons. Embo J. 2005;24(18):3224–34. [PMC free article: PMC1224685] [PubMed: 16121193]
- Determinants of 4 alpha-phorbol sensitivity in transmembrane domains 3 and 4 of the cation channel TRPV4.[J Biol Chem. 2007]Determinants of 4 alpha-phorbol sensitivity in transmembrane domains 3 and 4 of the cation channel TRPV4.Vriens J, Owsianik G, Janssens A, Voets T, Nilius B. J Biol Chem. 2007 Apr 27; 282(17):12796-803. Epub 2007 Mar 6.
- Cell swelling, heat, and chemical agonists use distinct pathways for the activation of the cation channel TRPV4.[Proc Natl Acad Sci U S A. 2004]Cell swelling, heat, and chemical agonists use distinct pathways for the activation of the cation channel TRPV4.Vriens J, Watanabe H, Janssens A, Droogmans G, Voets T, Nilius B. Proc Natl Acad Sci U S A. 2004 Jan 6; 101(1):396-401. Epub 2003 Dec 22.
- Review A New Insight into the Function of TRPV2 in Circulatory Organs.[TRP Ion Channel Function in Se...]Review A New Insight into the Function of TRPV2 in Circulatory Organs.Muraki K, Shigekawa M, Imaizumi Y. TRP Ion Channel Function in Sensory Transduction and Cellular Signaling Cascades. 2007
- Anandamide and arachidonic acid use epoxyeicosatrienoic acids to activate TRPV4 channels.[Nature. 2003]Anandamide and arachidonic acid use epoxyeicosatrienoic acids to activate TRPV4 channels.Watanabe H, Vriens J, Prenen J, Droogmans G, Voets T, Nilius B. Nature. 2003 Jul 24; 424(6947):434-8.
- Review TRPV4.[Handb Exp Pharmacol. 2007]Review TRPV4.Plant TD, Strotmann R. Handb Exp Pharmacol. 2007; (179):189-205.
- Molecular Mechanisms of TRPV4 Gating - TRP Ion Channel Function in Sensory Trans...Molecular Mechanisms of TRPV4 Gating - TRP Ion Channel Function in Sensory Transduction and Cellular Signaling Cascades
Your browsing activity is empty.
Activity recording is turned off.
See more...