NCBI Bookshelf. A service of the National Library of Medicine, National Institutes of Health.
Liedtke WB, Heller S, editors. TRP Ion Channel Function in Sensory Transduction and Cellular Signaling Cascades. Boca Raton (FL): CRC Press/Taylor & Francis; 2007.
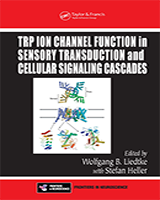
TRP Ion Channel Function in Sensory Transduction and Cellular Signaling Cascades.
Show detailsINTRODUCTION
Mammalian TRP channels form a large family with around thirty members. From sequence similarity, TRPs can be divided into three major TRP subfamilies: the classical or canonical subfamily (TRPC), the melastatin-related subfamily (TRPM), and the vanilloid-receptor–related subfamily (TRPV) [1]. In addition, there are a number of more distantly related subfamilies: TRPA (ankyrin), TRPP (polycystin), and TRPML (mucolipidin) [1–3].
TRPV1, the first member of the TRPV family and the sensory neuron receptor for vanilloid ligands like capsaicin, which is also responsive to noxious heat (>42°C), was found by expression cloning [4], as were the more distantly related epithelial Ca2+ channels TRPV5 [5] and TRPV6 [6]. The other members, TRPV2 [7], TRPV4 (see below), and later TRPV3 [8–10], were found by homology screens.
TRPV4 was found by screening expressed sequence tag databases for sequences with similarity to TRPV1, TRPV2, and the C. elegans TRPV isoform OSM-9. Lacking a consensus on nomenclature at the time, TRPV4 was given a variety of names—OTRPC4 (OSM-9-like TRP channel 4) [11], VROAC (vanilloid receptor–related osmotically activated channel) [12], TRP12 [13] and VRL-2 (vanilloid receptor–like channel 2) [14]—by the different groups who cloned the channel. TRPV4 has 871 amino acids, and structural features of the channel are intracellular N- and C-termini, six membrane spanning segments (S1–S6), a reentrant pore-forming loop between S5 and S6, and at least three ankyrin domains in the cytosolic N-terminus (see, e.g., Figure 9.2). Even though TRPV4 shows sequence similarity to other members of the TRPV family, particularly to TRPV1–3, a coexpression study has indicated that TRPV4 preferentially forms homomers [15], and, as yet, there is no evidence for heteromultimeric combinations with other TRPVs.
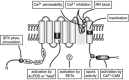
FIGURE 9.1
Responses of TRPV4-expressing HEK293 cells to the extracellular osmolarity and to the ligand 4αPMA. (A) [Ca2+]i measurements in TRPV4-expressing cells reveal an elevated basal [Ca2+]i in many cells indicative of spontaneous channel activity in (more...)
TISSUE EXPRESSION OF TRPV4
TRPV4 is widely expressed. TRPV4 is strongly expressed in the kidneys, and orthologues from most species (mouse, human, and rat) have been cloned from this tissue [11–14]. In addition, the human orthologue has been cloned from a hypothalamus library and the chicken variant from an auditory epithelium library [12].
In Northern hybridization, TRPV4 mRNA was detected in the heart, endothelium, brain, liver, placenta, lung, trachea, and salivary glands [11–14]. mRNA is also present in airway smooth muscle [16] and in the substantia nigra pars compacta [17]. In the brain, in situ hybridization shows obvious mRNA expression in neurons of the circumventricular organs and in ependymal cells of the choroid plexus of the lateral and fourth, but not third, ventricles, and in scattered neurons in other regions of the brain [12]. In the trigeminal ganglia and dorsal root ganglia, TRPV4 mRNA is present in large sensory neurons [12,14,18]. In the inner ear, TRPV4 mRNA is present in inner and outer hair cells of the organ of Corti, and in hair cells of the semicircular canals and utriculae [12]. TRPV4 mRNA is also present in the auditory ganglion and in marginal cells of the stria vascularis [12]. In the respiratory tract, TRPV4 protein is expressed in the epithelia of the trachea and lung, submucosal glands, and mononuclear cells [14]. Furthermore, protein was also detected in sympathetic ganglia and in sympathetic and parasympathetic nerve fibers in a number of tissues [14]. TRPV4 is also expressed in the hypothalamus and in keratinocytes [19], and in the epithelium of the oviduct [20]. In the kidney tubules, TRPV4 expression is localized to constitutively or conditionally water impermeable (antidiuretic hormone-sensitive) segments [14,21], where it is mainly localized to the basolateral membrane [21].
REGULATION OF TRPV4 ACTIVITY
Much of what is known about TRPV4 regulation comes from studies of the channel heterologously expressed in mammalian cells, although there are an increasing number of reports on a channel with similar properties in native tissues known to express TRPV4. Following heterologous expression, cells expressing TRPV4 often display spontaneous channel activity, which results in an elevated basal intracellular Ca2+ concentration ([Ca2+]i; Fig. 9.1A) and in spontaneous currents characteristic for TRPV4 (Fig. 9.1B,C). As yet unidentified intracellular factors are likely to be required for the maintenance of spontaneous channel activity as evidenced by the disappearance of currents within a few minutes in open whole cell patch-clamp (ruptured patch) recordings and the stable activity in perforated patch recordings. In the search for the mechanism of channel regulation, we tested a number of classical signaling pathways and cellular messengers but observed no clear increase or reduction in [Ca2+]i in cells expressing TRPV4 compared to control cells [11]. Unlike TRPV1, TRPV4 is insensitive to capsaicin [11].

FIGURE 9.2
Transmembrane topology of TRPV4 showing the sites shown to be involved in the regulation of the channel and in determining channel properties. Abbreviations: SFK: src family kinase, Ank: Ankryrin domain, RR: ruthenium red, CaMBD: calmodulin-binding domain. (more...)
Regulation of TRPV4 by the Extracellular Osmolarity
A number of cues indicated to investigators who first studied TRPV4 that it may be an osmotically or mechanically sensitive channel. These included the knowledge that OSM-9 from C. elegans is involved in behavioral responses to increases in extracellular osmolarity and mechanical stimulation in the worm [22]. Furthermore, some of the tissues where mammalian TRPV4 expression is found are exposed to changes in osmolarity (the kidney) or are involved in osmoregulation (the circumventricular organs) or mechanosensation (hair cells in the ear). Changes in osmolarity provide both an osmotic and mechanical stimulus, owing to cell swelling and membrane stretching.
TRPV4 is highly sensitive to changes in extracellular osmolarity around the normal osmolarity of body fluids. Reductions in the extracellular osmolarity result in increases in [Ca2+]i and membrane currents (Fig. 9.1A,B), whereas osmolarities above 300 mosmol l−1 decrease both [Ca2+]i and currents (Fig. 9.1C) in those cells displaying spontaneous activity [11]. Significant changes in Ca2+ influx were observed in response to changes in extracellular osmolarity of as little as 1 percent [12]. Stronger reductions in osmolarity result in larger increases in [Ca2+]i and currents, which are often not graded but show a slow increase followed by a very rapid response to a high level [11]. This behavior probably reflects the positive feedback effect of Ca2+ entry on the channel (see below). In addition to relatively stable increases in [Ca2+]i Liedtke et al. also reported that some cells respond to decreases in osmolarity with Ca2+ oscillations [12].
Responses to reduced osmolarity show differences depending on the expression system, recording conditions, and laboratory. Gao et al. [23] reported no elevation of basal Ca2+ in TRPV4-expressing cells and found very poor Ca2+ responses to hypotonic solutions at room temperature. Responses were nearly fourfold larger at 37°C. Others have observed strong [Ca2+]i responses to reduced osmolarity at room temperature [11,24]. A more intact intracellular environment is probably important for the response to hypotonicity. Current responses to hypotonic solutions are slow and weak in ruptured patch whole-cell recordings compared to those in perforated-patch recordings. In ruptured patch-clamp recordings, current responses to hypotonic solutions were also much smaller (ninefold) than responses to 4α-phorbol esters, potent ligands that activate TRPV4 [25]. Other groups report no response of TRPV4 to reduced osmolarity in CHO cells, but responses in HEK cells [26]. Other factors that may influence the response to changes in osmolarity include proteins like microtubule-associated protein 7 (MAP7) that interact with TRPV4 [27].
Consistent with the data on the osmosensitivity of heterologously expressed TRPV4, TRPV4−/− mice displayed defects in osmoregulation [28,29]. TRPV4 has also been suggested to be involved in the nociceptive response of primary sensory neurons [18,30], and the [Ca2+]i response of airway smooth muscle cells to hypotonic stimulation [16]. In endothelial cells, part of the response to hypotonic solutions is abolished in TRPV4−/− mice [31]. In C. elegans lacking the TRPV isoform OSM-9, TRPV4 targeted to the appropriate sensory neurons restored responses to osmotic stimuli [32]. Surprisingly, in this context, TRPV4 rescues responses to hypertonic solutions, whereas in mammalian cells, channel activation is seen on application of hypotonic solutions. The reason for this difference is unclear. Perhaps worm neurons, in contrast to mammalian cells, can generate an appropriate messenger to activate TRPV4 in response to hypertonic stimuli.
Mechanosensitivity of TRPV4
The involvement of TRPV4 in responses to cell swelling and its localization in tissues like the inner ear, sensory neurons, and endothelial cells that are known to express mechanosensitive channels raise the question of whether TRPV4 is a mechanosensitive channel. We saw no activation of TRPV4 in response to membrane stretch by applying suction to the patch pipette in the cell-attached mode in HEK293 cells [11]. A more recent study has, however, shown that TRPV4-expressing cells respond in the whole-cell mode to cell inflation with a current activation [26].
TRPV4’s responsiveness to noxious mechanical stimuli has also been suggested from a study of TRPV4−/− mice [26,28]. TRPV4 also rescues mechanosensitivity in sensory neurons from C. elegans lacking OSM-9 [32]. TRPV4 was a major candidate for a mechanosensitive channel in hair cells in the cochlea involved in hearing. TRPV4−/− mice displayed a delayed onset hearing loss and an increased susceptibility to acoustic injury [33]. The precise role of TRPV4 in hearing has not been identified, but the channel does not appear to be the mechanosensitive channel in hair cells.
The expression of TRPV4 in tissues that show flow- or shear stress-dependent Ca2+ entry like flow-sensitive nephron segments and the vascular endothelium make TRPV4 a candidate for a channel sensitive to these stimuli. Increases in [Ca2+]i sensitive to ruthenium red, an inhibitor of TRPV channels (see below), have been reported in response to shear stress in HEK293 cells expressing TRPV4 at 37°C, but not at room temperature [23]. To date, there has been no demonstration of TRPV4 involvement in responses to these stimuli in native tissues. An involvement in flow-sensitive Ca2+ increases in the nephron is not consistent with a report of a mainly basolateral localization in tubule cells [21]. Surprisingly, increases in viscosity produced by adding dextran to the extracellular solution have been reported to increase currents in Hela cells expressing TRPV4 [20].
Mechanism of Activation by Hypotonic Solutions and Mechanical Stimuli
In the original demonstrations that TRPV4 could be regulated by changes in the extracellular osmolarity, it was unclear how the channel responds to these changes. We were careful to point out that the channel may respond directly to changes in osmolarity or may be activated by an endogenous signaling cascade sensitive to swelling [11]. A delay of at least twenty seconds before TRPV4-expressing cells respond to osmotic stimuli, however, suggests that a slower process (e.g., cell swelling or signaling pathways activated by cell swelling) is involved in the response. Because many cellular signaling cascades have been demonstrated to be activated by changes in cell volume, there is no lack of prospective candidates [34]. In contrast to volume-regulated anion channels, which are activated by a mechanism involving a swelling-induced reduction in intracellular ionic strength, and tyrosine kinase activation via small GTP-binding proteins, TRPV4 did not respond to infusion of GTPγS or to reductions in intracellular ionic strength [35]. Nonreceptor tyrosine kinases have, however, been implicated in the response of TRPV4 to hypotonic solutions, and hypotonic stress has been reported to increase tyrosine phosphorylation of native and heterologously expressed TRPV4 by members of the Src family of tyrosine kinases [36]. When coexpressed with TRPV4, Lyn, a tyrosine kinase of this family, dramatically increased phosphorylation of Tyr253 (Figure 9.2) in response to treatment with hypotonic solutions [36]. Mutation of this tyrosine resulted in a loss of the Ca2+ response of TRPV4-expressing cells to hypotonic solutions, leading to the suggestion that the response of TRPV4 to hypotonic cell swelling is mediated by tyrosine kinase-dependent phosphorylation. However, neither Vriens et al. [24] nor we (unpublished data) could confirm the loss of responsiveness to hypotonic solutions with this mutant.
Watanabe et al. showed that TRPV4 can be activated by products of arachidonic acid breakdown [37]. Arachidonic acid and endogenous activators of TRPV4, like the cannabinoid anadamide, that yield arachidonic acid are metabolized in a cytochrome P450 epoxygenase-dependent manner to epoxyeicosatrienoic acids (EETs). Inhibition of the cytochrome P450 epoxygenase strongly inhibited channel activation by arachidonic acid, and application of EETs activated heterologously expressed and endothelial TRPV4 [31,37]. Responses to hypotonic solutions were reduced by structurally unrelated inhibitors of PLA2 and by inhibitors of CYP450 epoxygenase [24,31] and were increased by upregulation of the CYP450 epoxygenase [31]. From these data, it is probable that the sensitivity to changes in extracellular osmolarity that TRPV4 confers on cells results from activation of an endogenous signaling cascade activated by changes in cell volume: swelling-induced activation of PLA2, release of arachidonic acid from membrane phospholipids, followed by CYP450 epoxygenase-dependent metabolism of arachidonic acid to EETs, which then activate the channel. There is also evidence that, like the effects of hypotonic solutions, the activation of TRPV4 by increased extracellular viscosity is also PLA2 dependent [20]. However, it remains unclear whether EETs activate TRPV4 by a direct interaction with the channel or whether other mediators are involved.
A further interesting open question is how basal channel activity is generated and how reductions in [Ca2+]i and currents occur in response to hypertonic solutions. A simple explanation would be that PLA2 is active under isotonic conditions and that activity can be decreased by hypertonic solutions. However, Vriens et al. found that inhibitors of PLA2 have no effect on the basal activity of TRPV4 [24]. Thus, different mechanisms are likely to be involved in the generation of basal TRPV4 activity and in the response of the channel to hypotonic solutions.
Activation of TRPV4 by Phorbol Ester Derivatives
A very important finding for TRPV4 was the identification of phorbol ester derivatives as channel activators (Figure 9.1D) [25]. TRPV4 was found to be activated by 4α-phorbol ester derivatives with EC50 values around 0.2 μM for 4α-phorbol dide-canoate (4αPDD). These 4α-phorbol ester derivatives are commonly used as negative controls for the 4β-phorbol esters. The latter are widely used as activators of protein kinase C, (PKC) but also affect other proteins with C1-domains independently of PKC. The 4α-phorbol ester derivatives are not known to activate other ion channels and therefore represent specific tools to activate TRPV4 and study TRPV4-mediated effects. Under the conditions used in the study of Watanabe et al., the 4β-phorbol ester phorbol myristate acetate (PMA) was a less potent activator of TRPV4 than the 4α derivate, and acted as a partial agonist [25]. In ruptured patch whole-cell experiments, 4α-phorbol esters are more effective than hypotonic solutions in activating TRPV4 [25], but in more intact cells, [Ca2+]i increases in response to hypotonic solutions and 4αPDD are similar [24]. The effects of 4α-phorbol ester derivatives are not mediated by the PLA2 pathway that is involved in activating TRPV4 by hypotonic solutions, because 4α-phorbol ester-mediated activation still occurs in the presence of PLA2 inhibitors [24]. However, although the effects of hypotonic solutions and phorbol esters are mediated by different pathways, the effects of heat (see below) and 4αPDD seem to share a common mechanism. By analogy to TRPV1, for which a YS motif in the S2–S3 linker was shown to be involved in the binding of capsaicin [38], Vriens et al. identified a YS motif at the N-terminal end of S3 (Tyr555 and Ser556, Figure 9.2) [24]. Mutations of Tyr555 led to a loss of responsiveness to 4αPDD, but not to arachidonic acid, lending further support to the hypothesis that different pathways are involved in the responses to cell swelling and phorbol esters. Furthermore, the Tyr555 mutation also led to a loss of the response to heat (see below). The independence of pathways for the response of TRPV4 to cell swelling and arachidonic acid metabolism, and the responses to 4α-phorbol esters and heat have recently been confirmed in endothelial cells [31].
Studies performed at 37°C rather than at room temperature report different effects of 4β-phorbol ester derivatives [23,39]. At the higher temperatures, 4β-phorbol ester derivatives had larger effects than at room temperature, and a large part of these effects was prevented by PKC inhibitors. These results suggest that the 4β-phorbol ester derivatives may have PKC-dependent and PKC-independent effects on TRPV4.
Regulation of TRPV4 by Temperature
Members of the TRPV family, with the exception of TRPV5 and TRPV6, form temperature-sensitive channels with temperature sensitivities ranging from warm (TRPV3/TRPV4) through hot (TRPV1), to very hot (TRPV2) [40]. TRPV4 has a temperature activation threshold between 25°C and 33°C in HEK293 cells [19,41], and around 27°C in Xenopus oocytes [19]. Responses to warming show desensitization, but this is incomplete, leaving a spontaneously active current component at temperatures around 37°C. TRPV4 responses to heat are also observed in cell-attached patches, but not in cell-free patches [41], suggesting that cellular components not present or functional in excised patches are involved in the production of a messenger responsible for the heat response. Heat-activated currents are smaller than those in response to 4αPDD. The current density in response to warming to 38°C was about one fifth of that in response to 4αPDD. However, there seem to be similarities in the responses to heat and 4αPDD because both responses are lost in the mutants of the YS motif in S3 [24]. This suggests that heat may influence the production of an as-yet-unidentified endogenous messenger that binds to a region of TRPV4 involving S3.
Responses of TRPV4 to warm temperatures and its expression in sensory neurons, keratinocytes, and in the hypothalamus indicate a role for TRPV4 in thermosensation and thermoregulation. TRPV4−/− mice show decreased frequencies of nerve discharge in response to thermal stimulation, a decrease in the number of responsive fibers, and longer latencies to escape from thermal stimuli in a model of thermal hyperalgesia [42]. A lack of TRPV4 has no effect on escape latency in response to heat in the absence of hyperalgesia [26,28,42], with the exception of a study that described an increase in tail withdrawal latency to moderately hot temperatures [43]. The latter study also showed that TRPV4−/− mice showed a preference for warmer floor temperatures. TRPV4 expression in the hypothalamus is an indicator of a possible role of TRPV4 in thermoregulation. However, Liedtke and Friedman found no difference in body temperature or differences in the temperature response to cold stress in TRPV4−/− mice [28].
Ca2+ Dependence of TRPV4
Most Ca2+-permeable channels show some feedback regulation by Ca2+ to prevent deleteriously large increases in [Ca2+]i or to shape the time course of channel activity. There is clear experimental evidence that, in addition to allowing Ca2+ to permeate, TRPV4 is closely regulated by [Ca2+]i. As described above, responses of TRPV4 to hypotonic solutions, phorbol esters, or heat are transient with rapid activation followed by inactivation/decay (Figures 9.1B, D). Ca2+ is involved both in the decaying phase and the activation phase of TRPV4; in the latter, it is not only a charge carrier, but also a potentiator of channel activity.
Outward currents mediated by TRPV4 are strongly reduced by removing extracellular Ca2+. Similarly, removing extracellular Ca2+ abolishes spontaneous TRPV4 currents in parallel with the reduction in [Ca2+]i [44]. There is a very close correlation between [Ca2+]i and the amplitude of TRPV4 currents. Replacement of extracellular Ca2+ by Ba2+ or Sr2+, which also permeate well (see below), leads to a reduction in spontaneous inward and outward currents, a result that indicates that these ions are unable to substitute for Ca2+ in the mechanism involved in generating spontaneous channel activity. In the absence of extracellular Ca2+, or when Ca2+ is replaced by Ba2+, activation of TRPV4 by hypotonic solutions or 4αPMA is slow, taking several minutes rather than seconds to reach a maximum.
Ca2+ could either regulate the activity of TRPV4 by influencing Ca2+-dependent steps in signaling pathways leading to channel activation, or by binding to the channel or to channel-associated proteins. TRPV4 does not have an obvious Ca2+-binding site like an EF hand, suggesting that Ca2+ does not bind directly to the channel. Many other TRP channels have calmodulin (CaM)-binding sites in their C-termini, but regulation via Ca2+ and CaM has only been convincingly demonstrated for a few isoforms, including the TRPV isoforms TRPV1 [45] and TRPV6 [46,47]. We identified a CaM-binding site similar to that in the C-terminus of human TRPV6 [46] in the intracellular C-terminus of TRPV4 (Figure 9.2) [44]. This binding site is a highly conserved helical stretch of amino acids, VGRLRRDRWSSVVPRV, starting at position 814.
In in vitro CaM-binding experiments, mutations of amino acids within this region, including the point mutation W822A, prevented Ca2+-dependent CaM binding [44]. For the C-terminal CaM-binding site of TRPV6, similar mutations that prevent Ca2+-dependent CaM binding decrease the rate of a slower phase of channel inactivation [46]. In strong contrast to TRPV6, we found that the mutations that prevent CaM binding to C-terminal peptides of TRPV4 influence Ca2+-dependent potentiation and not inactivation of the channel.
Wild-type TRPV4 shows strong potentiation on the addition of Ca2+ following channel activation by hypotonic solutions or 4α-phorbol esters in a Ca2+-free medium [44]. After transient expression, the full-length channels with mutations that prevent Ca2+-CaM binding showed no potentiation of current responses to 4αPMA on Ca2+ addition, and only slow activation by 4αPMA in the presence of extracellular Ca2+. The speed of activation in Ca2+ resembled that of TRPV4-wt in the absence of Ca2+ or when Ba2+ replaced Ca2+.
From these data, we suggest that there is a Ca2+-dependent component of TRPV4 activation. Irrespective of the primary activator, hypotonic medium, lipophilic ligands, or heat, Ca2+ entering through TRPV4 binds to CaM, and Ca2+-CaM interacts with the C-terminal binding domain, resulting in positive feedback activation of TRPV4, increasing both the speed and amplitude of the response. It remains unclear, however, how Ca2+-CaM binding exerts its effects on channel activity and what the physiological role of this mechanism may be.
If unchecked, positive feedback involving Ca2+ could lead to large, potentially harmful increases in [Ca2+]i and, thus, some kind of inhibitory mechanism is necessary to turn the channel off. Ca2+ limits the entry of Ca2+ through other Ca2+-permeable channels by feedback inhibition. Likewise, Ca2+ is involved in the decay of TRPV4 currents and in controlling channel availability. Large, fast TRPV4 current responses decay rapidly to levels below those of spontaneous currents before activation (see, e.g., Figure 9.1B), even in the continuous presence of the activating stimulus. Current responses in the absence of Ca2+ are in general smaller, and display slower activation kinetics (as described above) but also much weaker current decay, even when current amplitudes similar to those in the presence of Ca2+ are attained. With increasing extracellular [Ca2+], current responses to 4α-phorbol esters activate more rapidly (as expected from the Ca2+-dependent potentiation described above), become smaller in amplitude, and decay more completely and rapidly [48]. Watanabe et al. [48] also saw a reduction in current amplitude as [Ca2+]i was raised via the intracellular solution in the patch pipette, with an IC50 of around 600 nM.
The mechanism by which Ca2+ inactivates TRPV4 remains unclear. Other TRPV channels (TRPV1 and TRPV6) have been shown to inactivate in a Ca2+-dependent fashion. TRPV6 shows biphasic inactivation kinetics, with a fast component in the millisecond and a slow component in the second range. The fast component was shown to depend on a sequence motif in the first intracellular loop [49], while the slow component is mediated by Ca2+-CaM binding to a C-terminal binding domain [46]. Both sites have no homologues in the TRPV4 sequence.
By analogy to TRPV1, however, for which a tyrosine residue in S6 has been shown to be involved in Ca2+ permeation and Ca2+-dependent desensitization [50], Watanabe et al. found that mutation of a phenylalanine residue at position 707 in S6 of TRPV4 (Figure 9.2) to alanine resulted in a reduction and slowing of current decay but did not modify the sensitivity to [Ca2+]i. [48] In the same study, mutation of Glu797 in the C-terminal tail (Figure 9.2) increased spontaneous channel activity, but the increase in [Ca2+]i in response to 4αPDD was similar [48]. Glu797 is close to the CaM-binding domain, and it remains to be seen whether the mutation of this residue influences CaM binding. Thus, Ca2+-dependent inactivation of TRPV4 seems to rely on structural features that have not yet been identified.
Interaction of Different Stimuli
Because a number of mechanisms converge on TRPV4, they are likely to interact and influence one another. Liedtke et al. found that responses of TRPV4 to decreases in osmolarity were larger at 37°C than at room temperature [12]. As described above for the individual activators, Gao et al. found that responses to most activators, including 4αPDD, PMA, hypotonic solutions, and shear stress, are increased at 37°C compared to room temperature [23]. For the response of TRPV4 to temperature, raising the osmolarity very significantly depressed responses to heat in HEK293 cells and oocytes, whereas decreasing the osmolarity initiated a response alone and potentiated the response to heat in HEK293 cells [19]. Somewhat surprisingly, however, the effects of 5,6-EETs, unlike the effects of hypotonic solutions [12,23], were found not to be affected by heat and were reduced in hypoosmotic conditions [51].
PROPERTIES OF CHANNELS FORMED BY TRPV4
Biophysical Properties
TRPV1 to TRPV4 differ not only in their sequences but also in their biophysical properties from TRPV5 and TRPV6. Many of the differences in properties result from differences in the Ca2+ permeability of the isoforms. The IV relation of TRPV4 has a characteristic shape, showing outward rectification in Ca2+-containing solutions, but also an increase in conductance, and concomitantly in inward current, at negative membrane potentials (Figure 9.1B, C, D). The reversal potential in normal extracellular solutions is just positive to 0 mV (Figure 9.1C, D). The outward rectification results from a block by extracellular Ca2+. Lowering the extracellular [Ca2+] increases inward currents and leads to a loss of rectification in Ca2+-free solutions [52]. Asp672 and Asp682 in the pore region (Figure 9.2) are involved in the inhibition of TRPV4 currents by extracellular Ca2+. Neutralization of both leads to a strong reduction in inhibition and a linearization of the IV relation [52]. Interestingly, the IV shape is similar for single-channel and whole-cell currents. Because of the outward rectification, the single-channel conductance for outward currents (88–100 pS) is larger than that for inward currents (30–60 pS). A value of 310 pS at +80 mV, a value much larger than that in other studies, was obtained in a cursory investigation of single-channel properties in another study [12]. In studies other than the latter, the relatively large variability in the conductance values obtained for inward currents probably results from differences in the solutions bathing the external face of the patch. Lower values were obtained in more physiological Ca2+ concentrations [11] than in Ca2+-free solutions [37,41], as expected from the effect of Ca2+ on whole-cell currents [52]. Thus, where studied carefully, data on single-channel conductance are consistent, and variabilities in the conductance for inward currents result from the use of different experimental conditions.
The reversal potential of around +5 to +10 mV for TRPV4 with standard extra-and intracellular solutions and abolition of most of the inward current on replacement of extracellular cations with the large cation N-methyl-D-glucamine (NMDG+) indicates that the channel is a nonselective cation channel. TRPV4 is moderately Ca2+ permeable with PCa/PNa values of between 6 and 10 [11,25]. Other divalent cations alsopermeate with a PMg/PNa of 2–3, a PSr/PNa of 9, and PBa/PNa a of 0.7–7 [25,44,52]. The channel discriminates poorly among monovalent cations with PK:PCs:PNa:PLi = 2:1.3:1:0.9 [25,35]. Mutations that affect Ca2+ inhibition and the shape of the IV relation also moderately reduce the Ca2+ permeability [52]. Mutation of Met680, which, by analogy to K+ channels, is located at the center of the selectivity filter (Figure 9.2), leads to a reduction in current and a strong reduction in Ca2+ permeability [52].
Blockers
Studies on trp channels suffer from the lack of specific pharmacological modulators. Like other members of the TRPV subfamily, TRPV4 is blocked by extracellular ruthenium red (RR) in micromolar concentrations [11,25,52]. The block by RR is voltage dependent, with inward currents being more strongly inhibited than outward currents. It is also notable that inward currents are inhibited much more rapidly than outward currents. For native currents mediated by TRPV4 in keratinocytes, but not in HEK293 cells, an increase in outward current on RR application has been reported [53]. In our hands, application of 10 μM RR to TRPV4 in HEK293 cells resulted in an increase in outward current followed by inhibition (unpublished observations). Neutralization of Asp682 (Figure 9.2), a residue involved in the block of TRPV4 by extracellular Ca2+ (see above), shifted the potential dependence of and slowed the block by RR [52]. Thus, Asp682 is likely to be in the outer region of the pore accessible to the large cation. TRPV4 is also inhibited by micromolar Gd3+11 and SKF96365 [32], which, however, inhibit many other TRP channels and native cation channels in a similar concentration range and cannot be considered specific.
CONCLUSIONS
A number of the TRPV channels are likely to have highly specific roles, and their expression is restricted to a very limited number of tissues. TRPV4 shows a wider expression pattern, with expression in cells with a variety of physiological functions from sensory transduction to epithelial transport. In addition, TRPV4 shares with TRPV1 the property of being regulated by a surprising variety of stimuli. For TRPV4, these include physical stimuli like osmotic stress, mechanical stress, and heat, as well as endogenous lipid agonists. Unlike many channels that serve the same specific functional role in different tissues, data emerging for TRPV4 suggest that the physiological stimulus may depend on the tissue context and that a combination of stimuli will determine the activity of the channel.
REFERENCES
- 1.
- Clapham DE, Montell C, Schultz G, Julius D. International Union of Pharmacology. XLIII. Compendium of voltage-gated ion channels: transient receptor potential channels. Pharmacol Rev. 2003;55:591–596. [PubMed: 14657417]
- 2.
- Moran MM, Xu H, Clapham DE. TRP ion channels in the nervous system. Curr Opin Neurobiol. 2004;14:362–369. [PubMed: 15194117]
- 3.
- Pedersen SF, Owsianik G, Nilius B. TRP channels: An overview. Cell Calcium. 2005;38:233–252. [PubMed: 16098585]
- 4.
- Caterina M, Schumacher MA, Tominaga M, Rosen TA, Levine JD, Julius D. The capsaicin receptor: a heat-activated ion channel in the pain pathway. Nature. 1997;389:816–824. [PubMed: 9349813]
- 5.
- Hoenderop JGJ, van der Kemp AWCM, Hartog A, van de Graaf SFJ, van Os CH, Willems PHGM, Bindels RJM. Molecular identification of the apical Ca2+ channel in 1,25-dihydroxyvitamin D3-responsive epithelia. J Biol Chem. 1999;274:8375–8378. [PubMed: 10085067]
- 6.
- Peng JB, Chen XZ, Berger UV, Vassilev PM, Tsukaguchi H, Brown EM, Hediger MA. Molecular cloning and characterization of a channel-like transporter mediating intestinal calcium absorption. J Biol Chem. 1999;274:22739–22746. [PubMed: 10428857]
- 7.
- Caterina MJ, Rosen TA, Tominaga M, Brake AJ, Julius D. A capsaicin receptor homologue with a high threshold for noxious heat. Nature. 1999;398:436–441. [PubMed: 10201375]
- 8.
- Smith GD, Gunthorpe MJ, Kelsell RE, Hayes PD, Reilly P, Facer P, Wright JE, Jerman JC, Walhin JP, Ooi L, Egerton J, Charles KJ, Smart D, Randall AD, Anand P, Davis JB. TRPV3 is a temperature-sensitive vanilloid receptor-like protein. Nature. 2002;418:186–190. [PubMed: 12077606]
- 9.
- Xu H, Ramsey IS, Kotecha SA, Moran MM, Chong JA, Lawson D, Ge P, Lilly J, Silos-Santiago I, Xie Y, DiStefano PS, Curtis R, Clapham DE. TRPV3 is a calcium-permeable temperature-sensitive cation channel. Nature. 2002;418:181–186. [PubMed: 12077604]
- 10.
- Peier AM, Reeve AJ, Andersson DA, Moqrich A, Earley TJ, Hergarden AC, Story GM, Colley S, Hogenesch JB, McIntyre P, Bevan S, Patapoutian A. A heat-sensitive TRP channel expressed in keratinocytes. Science. 2002;296:2046–2049. [PubMed: 12016205]
- 11.
- Strotmann R, Harteneck C, Nunnenmacher K, Schultz G, Plant TD. OTRPC4, a nonselective cation channel that confers sensitivity to extracellular osmolarity. Nat Cell Biol. 2000;2:695–702. [PubMed: 11025659]
- 12.
- Liedtke W, Choe Y, Marti-Renom MA, Bell AM, Denis CS, Sali A, Hudspeth AJ, Friedman JM, Heller S. Vanilloid receptor-related osmotically activated channel (VR-OAC), a candidate vertebrate osmoreceptor. Cell. 2000;103:525–535. [PMC free article: PMC2211528] [PubMed: 11081638]
- 13.
- Wissenbach U, Bödding M, Freichel M, Flockerzi V. Trp12, a novel Trp-related protein from kidney. FEBS Lett. 2000;485:127–134. [PubMed: 11094154]
- 14.
- Delany NS, Hurle M, Facer P, Alnadaf T, Plumpton C, Kinghorn I, See CG, Costigan M, Anand P, Woolf CJ, Crowther D, Sanseau P, Tate SN. Identification and characterization of a novel human vanilloid receptor-like protein, VRL-2. Physiol Genomics. 2001;4:165–174. [PubMed: 11160995]
- 15.
- Hellwig N, Albrecht N, Harteneck C, Schultz G, Schaefer M. Homo- and heteromeric assembly of TRPV channel subunits. J Cell Sci. 2005;118:917–928. [PubMed: 15713749]
- 16.
- Jia Y, Wang X, Varty L, Rizzo CA, Yang R, Correll CC, Phelps PT, Egan RW, Hey JA. Functional TRPV4 channels are expressed in human airway smooth muscle cells. Am J Physiol Lung Cell Mol Physiol. 2004;28:7, L272–278. [PubMed: 15075247]
- 17.
- Guatteo E, Chung KK, Bowala TK, Bernardi G, Mercuri NB, Lipski J. Temperature sensitivity of dopaminergic neurons of the substantia nigra pars compacta: involvement of TRP channels. J Neurophysiol. 2005;94:3069–3080. [PubMed: 16014800]
- 18.
- Alessandri-Haber N, Yeh JJ, Boyd AE, Parada CA, Chen X, Reichling DB, Levine JD. Hypotonicity induces TRPV4-mediated nociception in rat. Neuron. 2003;39:497–511. [PubMed: 12895423]
- 19.
- Güler AD, Lee H, Iida T, Shimizu I, Tominaga M, Caterina M. Heat-evoked activation of the ion channel, TRPV4. J Neurosci. 2002;22:6408–6414. [PMC free article: PMC6758176] [PubMed: 12151520]
- 20.
- Andrade YN, Fernandes J, Vazquez E, Fernandez-Fernandez JM, Arniges M, Sanchez TM, Villalon M, Valverde MA. TRPV4 channel is involved in the coupling of fluid viscosity changes to epithelial ciliary activity. J Cell Biol. 2005;168:869–874. [PMC free article: PMC2171792] [PubMed: 15753126]
- 21.
- Tian W, Salanova M, Xu H, Lindsley JN, Oyama TT, Anderson S, Bachmann S, Cohen DM. Renal expression of osmotically responsive cation channel TRPV4 is restricted to water-impermeant nephron segments. Am J Physiol Renal Physiol. 2004;28:7, F17–24. [PubMed: 15026302]
- 22.
- Colbert HA, Smith TL, Bargmann CI. Osm-9, a novel protein with structural similarity to channels, is required for olfaction, mechanosensation and olfactory adaptation in Caenorhabditis elegans. J Neurosci. 1997;17:8259–8269. [PMC free article: PMC6573730] [PubMed: 9334401]
- 23.
- Gao X, Wu L, O’Neil RG. Temperature-modulated diversity of TRPV4 channel gating: activation by physical stresses and phorbol ester derivatives through protein kinase C-dependent and -independent pathways. J Biol Chem. 2003;278:27129–27137. [PubMed: 12738791]
- 24.
- Vriens J, Watanabe H, Janssens A, Droogmans G, Voets T, Nilius B. Cell swelling, heat, and chemical agonists use distinct pathways for the activation of the cation channel TRPV4. Proc Natl Acad Sci USA. 2004;101:396–401. [PMC free article: PMC314196] [PubMed: 14691263]
- 25.
- Watanabe H, Davis JB, Smart D, Jerman JC, Smith GD, Hayes P, Vriens J, Cairns W, Wissenbach U, Prenen J, Flockerzi V, Droogmans G, Benham CD, Nilius B. Activation of TRPV4 channels (hVRL-2/mTRP12) by phorbol derivatives. J Biol Chem. 2002;277:13569–13577. [PubMed: 11827975]
- 26.
- Suzuki M, Mizuno A, Kodaira K, Imai M. Impaired pressure sensation in mice lacking TRPV4. J Biol Chem. 2003;278:22664–22668. [PubMed: 12692122]
- 27.
- Suzuki M, Hirao A, Mizuno A. Microtubule-associated protein 7 increases the membrane expression of transient receptor potential vanilloid 4 (TRPV4). J Biol Chem. 2003;278:51448–51453. [PubMed: 14517216]
- 28.
- Liedtke W, Friedman JM. Abnormal osmotic regulation in trpv4−/− mice. Proc Natl Acad Sci USA. 2003;100:13698–13703. [PMC free article: PMC263876] [PubMed: 14581612]
- 29.
- Mizuno A, Matsumoto N, Imai M, Suzuki M. Impaired osmotic sensation in mice lacking TRPV4. Am J Physiol Cell Physiol. 2003;28:5, C96–101. [PubMed: 12777254]
- 30.
- Alessandri-Haber N, Dina OA, Yeh JJ, Parada CA, Reichling DB, Levine JD. Transient receptor potential vanilloid 4 is essential in chemotherapy-induced neuropathic pain in the rat. J Neurosci. 2004;24:4444–4452. [PMC free article: PMC6729449] [PubMed: 15128858]
- 31.
- Vriens J, Owsianik G, Fisslthaler B, Suzuki M, Janssens A, Voets T, Morisseau C, Hammock BD, Fleming I, Busse R, Nilius B. Modulation of the Ca2+permeable cation channel TRPV4 by cytochrome P450 epoxygenases in vascular endothelium. Circ Res. 2005;97:908–915. [PubMed: 16179585]
- 32.
- Liedtke W, Tobin DM, Bargmann CI, Friedman JM. Mammalian TRPV4 (VR-OAC) directs behavioral responses to osmotic and mechanical stimuli in Caenorhabditis elegans. Proc Natl Acad Sci USA. 2003;100(Suppl 2):14531–14536. [PMC free article: PMC304114] [PubMed: 14581619]
- 33.
- Tabuchi K, Suzuki M, Mizuno A, Hara A. Hearing impairment in TRPV4 knockout mice. Neurosci Lett. 2005;382:304–308. [PubMed: 15925108]
- 34.
- Lang F, Busch GL, Ritter M, Volkl H, Waldegger S, Gulbins E, Haussinger D. Functional significance of cell volume regulatory mechanisms. Physiol Rev. 1998;78:247–306. [PubMed: 9457175]
- 35.
- Nilius B, Prenen J, Wissenbach U, Bödding M, Droogmans G. Differential activation of the volume-sensitive cation channel TRP12 (OTRPC4) and volume-regulated anion currents in HEK-293 cells. Pflügers Arch. 2001;443:227–233. [PubMed: 11713648]
- 36.
- Xu H, Zhao H, Tian W, Yoshida K, Roullet JB, Cohen DM. Regulation of a transient receptor potential (TRP) channel by tyrosine phosphorylation. SRC family kinase-dependent tyrosine phosphorylation of TRPV4 on TYR-253 mediates its response to hypotonic stress. J Biol Chem. 2003;278:11520–11527. [PubMed: 12538589]
- 37.
- Watanabe H, Vriens J, Prenen J, Droogmans G, Voets T, Nilius B. Anandamide and arachidonic acid use epoxyeicosatrienoic acids to activate TRPV4 channels. Nature. 2003;424:434–438. [PubMed: 12879072]
- 38.
- Jordt SE, Julius D. Molecular basis for species-specific sensitivity to ‘‘hot’’ chili peppers. Cell. 2002;108:421–430. [PubMed: 11853675]
- 39.
- Xu F, Satoh E, Iijima T. Protein kinase C-mediated Ca2+ entry in HEK 293 cells transiently expressing human TRPV4. Br J Pharmacol. 2003;140:413–421. [PMC free article: PMC1574039] [PubMed: 12970074]
- 40.
- Patapoutian A, Peier AM, Story GM, Viswanath V. ThermoTRP channels and beyond: mechanisms of temperature sensation. Nat Rev Neurosci. 2003;4:529–539. [PubMed: 12838328]
- 41.
- Watanabe H, Vriens J, Suh SH, Benham CD, Droogmans G, Nilius B. Heat-evoked activation of TRPV4 channels in an HEK293 cell expression system and in native mouse aorta endothelial cells. J Biol Chem. 2002;277:47044–47051. [PubMed: 12354759]
- 42.
- Todaka H, Taniguchi J, Satoh J, Mizuno A, Suzuki M. Warm temperature-sensitive transient receptor potential vanilloid 4 (TRPV4) plays an essential role in thermal hyperalgesia. J Biol Chem. 2004;279:35133–35138. [PubMed: 15187078]
- 43.
- Lee H, Iida T, Mizuno A, Suzuki M, Caterina MJ. Altered thermal selection behavior in mice lacking transient receptor potential vanilloid 4. J Neurosci. 2005;25:1304–1310. [PMC free article: PMC6725965] [PubMed: 15689568]
- 44.
- Strotmann R, Schultz G, Plant TD. Ca2+-dependent potentiation of the non-selective cation channel TRPV4 is mediated by a C-terminal calmodulin-binding site. J Biol Chem. 2003;278:26541–26549. [PubMed: 12724311]
- 45.
- Numazaki M, Tominaga T, Takeuchi K, Murayama N, Toyooka H, Tominaga M. Structural determinant of TRPV1 desensitization interacts with calmodulin. Proc Natl Acad Sci USA. 2003;100:8002–8006. [PMC free article: PMC164702] [PubMed: 12808128]
- 46.
- Niemeyer BA, Bergs C, Wissenbach U, Flockerzi V, Trost C. Competitive regulation of CaT-like-mediated Ca2+ entry by protein kinase C and calmodulin. Proc Natl Acad Sci USA. 2001;98:3600–3605. [PMC free article: PMC30699] [PubMed: 11248124]
- 47.
- Lambers TT, Weidema AF, Nilius B, Hoenderop JG, Bindels RJ. Regulation of the mouse epithelial Ca2+ channel TRPV6 by the Ca2+-sensor calmodulin. J Biol Chem. 2004;279(28):28855–28861. [PubMed: 15123711]
- 48.
- Watanabe H, Vriens J, Janssens A, Wondergem R, Droogmans G, Nilius B. Modulation of TRPV4 gating by intra- and extracellular Ca2+ Cell Calcium. 2003;33:489–495. [PubMed: 12765694]
- 49.
- Nilius B, Prenen J, Hoenderop JG, Vennekens R, Hoefs S, Weidema AF, Droogmans G, Bindels RJ. Fast and slow inactivation kinetics of the Ca2+channels ECaC1 and ECaC2 (TRPV5 and 6): role of the intracellular loop located between transmembrane segment 2 and 3. J Biol Chem. 2002;277:30852–30858. [PubMed: 12077127]
- 50.
- Mohapatra DP, Wang SY, Wang GK, Nau C. A tyrosine residue in TM6 of the vanilloid receptor TRPV1 involved in desensitization and calcium permeability of capsaicin-activated currents. Mol Cell Neurosci. 2003;23:314–324. [PubMed: 12812762]
- 51.
- Nilius B, Vriens J, Prenen J, Droogmans G, Voets T. TRPV4 calcium entry channel: a paradigm for gating diversity. Am J Physiol Cell Physiol. 2004;28:6, C195–205. [PubMed: 14707014]
- 52.
- Voets T, Prenen J, Vriens J, Watanabe H, Janssens A, Wissenbach U, Bödding M, Droogmans G, Nilius B. Molecular determinants of permeation through the cation channel TRPV4. J Biol Chem. 2002;277:33704–33710. [PubMed: 12093812]
- 53.
- Chung MK, Lee H, Mizuno A, Suzuki M, Caterina MJ. TRPV3 and TRPV4 mediate warmth-evoked currents in primary mouse keratinocytes. J Biol Chem. 2004;279:21569–21575. [PubMed: 15004014]
- Review Determining the Crystal Structure of TRPV6.[Calcium Entry Channels in Non-...]Review Determining the Crystal Structure of TRPV6.Saotome K, Singh AK, Sobolevsky AI. Calcium Entry Channels in Non-Excitable Cells. 2018
- Review TRP Channels and Pain.[Neurobiology of TRP Channels. ...]Review TRP Channels and Pain.González-Ramírez R, Chen Y, Liedtke WB, Morales-Lázaro SL. Neurobiology of TRP Channels. 2017
- Review Pharmacology of vanilloid transient receptor potential cation channels.[Mol Pharmacol. 2009]Review Pharmacology of vanilloid transient receptor potential cation channels.Vriens J, Appendino G, Nilius B. Mol Pharmacol. 2009 Jun; 75(6):1262-79. Epub 2009 Mar 18.
- Review TRP Channels: What Do They Look Like?[Neurobiology of TRP Channels. ...]Review TRP Channels: What Do They Look Like?Rosasco MG, Gordon SE. Neurobiology of TRP Channels. 2017
- Review Vanilloid and melastatin transient receptor potential channels in vascular smooth muscle.[Microcirculation. 2010]Review Vanilloid and melastatin transient receptor potential channels in vascular smooth muscle.Earley S. Microcirculation. 2010 May; 17(4):237-49.
- TRPV4: A Multifunctional Nonselective Cation Channel with Complex Regulation - T...TRPV4: A Multifunctional Nonselective Cation Channel with Complex Regulation - TRP Ion Channel Function in Sensory Transduction and Cellular Signaling Cascades
- Molecular Mechanisms of TRPV4 Gating - TRP Ion Channel Function in Sensory Trans...Molecular Mechanisms of TRPV4 Gating - TRP Ion Channel Function in Sensory Transduction and Cellular Signaling Cascades
Your browsing activity is empty.
Activity recording is turned off.
See more...