NCBI Bookshelf. A service of the National Library of Medicine, National Institutes of Health.
Mobley HLT, Mendz GL, Hazell SL, editors. Helicobacter pylori: Physiology and Genetics. Washington (DC): ASM Press; 2001.
Morphology of Helicobacter pylori
H. pylori, a dominant human pathogen, is now recognized as the first member of an ultrastructurally diverse genus. The predominant morphological form of this genus is curved to spiral; however, some members have either a short or tapered rod shape. All helicobacters are motile by means of flagella. These basic characteristics of morphology and motility are thought to be advantageous to these organisms due to their localization in the mucous layer of the gastrointestinal tracts of humans and a variety of animals. This chapter will concentrate on the ultrastructural features of H. pylori, with limited descriptions of some of the more unusual features seen in the other members of this genus. Certain aspects of the ultrastructural detail of the helicobacters, e.g., sheathed flagella and surface urease, have been implicated in their ability to survive in hostile environments such as the acidic gastric mucosa and their ability to induce disease.
Basic Morphology
H. pylori in vivo and under optimum in vitro conditions is an S-shaped bacterium with 1 to 3 turns, 0.5 ×5 μm in length, with a tuft of 5 to 7 polar sheathed flagella (40, 51). This morphology has been correlated with maximum in vitro motility (106). The majority of helicobacters possess this basic morphology of an S shape with polar, sheathed flagella, though variations in size and the number of spirals/turns are seen (Fig. 1). However, other variant forms are seen in a number of other species (80). Helicobacter mustelae is a short rod with lateral and polar flagella, whereas Helicobacter felis, Helicobacter bizzozeronii, Helicobacter suis, and "Helicobacter heilmannii" have a tight helical morphology and a characteristic corkscrew motility (Fig. 1). Helicobacter bilis, Helicobacter trogontum, and "Helicobacter rappini" have a fusiform shape, that is, a rod with tapered ends. Periplasmic fibers, which are clearly distinct from flagella, have been seen on H. felis, Helicobacter muridarum, H. bilis, H. trogontum, and "H. rappini" (Fig. 1). Two of the currently recognized genera, Helicobacter pullorum and Helicobacter rodentium, and a recently proposed new species, Helicobacter ganmani, all possess unsheathed flagella (B. Robertson, personal communication). Generally it is considered that the spiral morphology and flagella are essential for colonization of gastric and intestinal mucus (34).
Internal Organization
Thin sections of H. pylori reveal the typical cell wall detail of a gram-negative bacterium that consists of outer and inner, or plasma, membranes separated by the periplasm of approximately 30 nm thickness. The dense cytoplasm contains nucleoid material and ribosomes. Analysis of the peptidoglycan from H. pylori revealed it had a unique muropeptide composition, being less complex structurally than that seen in other gram-negative bacteria (26). In the majority of helicobacter species examined, an electron-lucent area is located in the terminal regions (57). Associated with this region and located near the flagella insertion site is a "polar membrane." This is an additional electron-dense band 6 to 8 nm thick located 20 nm below the plasmic membrane yet linked to it. Similar features have been described in a number of other genera including Spirillum and Campylobacter (37, 72). Brock and Murray showed the polar membrane to be an assembly of ATPase molecules probably located at this site to generate energy for motility or cell wall synthesis (19). Such a membrane was not seen in H. mustelae; instead, O'Rourke et al. identified a large electron-dense area of 80 ×100 nm adjacent to or below the flagella insertion point and proposed that this may perform a similar function to the polar membrane seen in the other helicobacters (81).
Intracellular granules have been observed in a number of gastric helicobacters including "H. heilmannii"-like bacteria and H. felis. However, Bode et al. first described them in detail in H. pylori and confirmed their identity as polyphosphate granules (17). Electron energy loss spectroscopy (EELS) identified a prominent phosphorus signal at three different locations: the cytoplasm, the flagella pole, and associated with the cell membrane. The granules (signal) located in the cytoplasm were the largest, 0.05 to 0.2 μm in size, amorphous and vacuole-like, and generally regarded as an energy source and phosphorus reservoir. Those located near the flagella pole were smaller, 0.02 μm in size, more compact and heterogeneous, and possibly provided energy required for motility of the cells. Specific staining with lead salts was required to detect the small granules, >0.01 μm in size, associated with the cell membrane that have been implicated in the maintenance of the cytoplasmic membrane.
Recently, studies of ferritin in H. pylori showed that in a wild-type strain grown in an iron-rich environment, cytoplasmic aggregates containing iron could be detected. No such structures were seen in a mutant that lacked the prf gene that encodes ferritin (12). Two forms of aggregates could be identified: large (0.05 to 2 μm), amorphous, vacuole-like aggregates containing iron, phosphorus, oxygen, and carbon and a smaller granular-like form (0.02 to 0.1 μm) that contained iron, oxygen, and carbon. It was proposed that ferritin was a major iron-containing component of the cytoplasm that could be used by the bacterium if required, such as to enable survival under harsh environmental conditions.
Cell Surface
Urease, GroEL analog, and other outer membrane proteins
Early studies by Jones et al. showed that after sarcosinate treatment, small "donut" structures, 12 nm in diameter with a 4-nm darkly staining central hole, could be seen (Fig. 2) (51). Subsequently it was shown from in situ studies and protein purification procedures that these structures represented cell surface-located urease, with evidence of shedding of this enzyme from the cell surface (16, 41). They have also been seen on H. mustelae (81). Examination of these "donuts" by negative staining and shadowing showed that they were macromolecular structures that stacked together in pairs, which again stacked together to form aggregates of four (9).

Figure 2
Surface structures of H. pylori. Negative stain of sarcosi-nate-treated bacteria showing urease as represented by the small donut-like structures (small arrow) and the flagella insertion base (large arrow), bar = 0.1 μm.
A second protein, identified as a GroEL homolog (Hp60K), has also been described. It is very similar in size to urease and appears as a 13-nm disc-like structure with a sevenfold rotational symmetry compared to a threefold symmetry seen with some urease particles. The Hp60K discs also formed regular stacks of four (10). By excluding flagellin, urease, and GroEL from their preparations, Doig and Trust were able to describe eight other outer membrane antigens of H. pylori, six of which were shown to be surface exposed. Two of the antigens were identified as lipopolysaccharides (LPS) that reacted with the outer membrane and flagella sheath and were found to vary in antigenic specificity depending on the strain of H. pylori examined. Three of the remaining antigens were thought to be porin proteins, with no ascertainable function assigned to the remaining three proteins (31).
Vacuolating cytotoxin
The vacuolating cytotoxin (VacA) of H. pylori has been associated with a >600-kDa protein consisting of a number of 95-kDa polypeptides that are formed from two subunits 37 and 58 kDa in size. Electron microscopic studies showed the cytotoxin resembled a flower with six or seven petals, 5 to 6 nm in diameter, with a clockwise cant and a central ring cavity of 12 to 15 nm, to give an overall diameter of 30 nm (27, 64). Two forms, one with a raised central ring and a flatter form, were observed. Lupetti et al. proposed that this was due to the loss of the central ring structures after proteolysis (64). However, this was later challenged by Cover et al. (27). In the latter report, it was found that after acid treatment the cytotoxin consisted of up to 12 petals with no central ring or a number of scattered petals. These structures could reassociate into the six- to seven-petal flowerlike formation after neutralization. Cover et al. proposed that the VacA cytotoxin was composed of 12~90-kDa subunits that associated to form a dodecamer. The dodecamer consisted of two 6-membered arrays that had two distinct faces, one flat and one with a raised central ring. These two distinct forms oriented face to face.
Similarities were drawn by both groups of the resemblance of this cytotoxin to A-B toxins seen in a number of other bacteria. With these toxins the B subunit binds to the cellular membrane, resulting in the formation of an ion channel that allows for the internalization of the active A subunit. Whether this is an appropriate model and whether VacA requires acid activation need further clarification.
Flagella and Periplasmic Fibers
All helicobacters are motile by means of flagella, which in most cases are sheathed, with configurations ranging from a single polar flagellum through to bipolar tufts of up to 20 flagella. Ultrastructurally, flagella have been shown to be composed of a basal body consisting of a rod and four concentric rings located in the various layers of the cell wall complex, a hook that in turn connects to a filament (Fig. 1b) (30). The flagellum of H. pylori is 30 nm in diameter, consisting of an internal filament ~12 nm in diameter surrounded by a sheath, the outer membrane of which is continuous with the outer membrane of the cell (40, 51). A terminal bulb is seen on many flagella that is possibly an artificat due to fixation. A 90-nm disc, thought to be the basal body of the flagellum, can be clearly seen on negatively stained preparations of the flagella insertion points. This disc can be separated by sarcosinate treatment and is degenerated by trypsin (51). Freeze-fracture preparations of the insertion points revealed a convex structure surrounded by a regular array to form what has been termed a "stud" and seen in many multiflagellated bacteria (72).
Characterization of the proteins associated with the flagella components indicate the hook protein (FlgE) is 78 kDa in size while the filament consists of two flagellin proteins, FlaA (53 kDa) and FlaB (54 kDa). Mutants lacking the flgE gene were nonmotile and lacked filaments; however, flagellins were still produced (82). Immunoelectron microscopic studies showed FlaB to be located close to the hook, with the dominant FlaA comprising the rest of the filament (54). Both flagellin subunits have been shown to be required for motility and the ability to persistently colonize gastric mucosa (52). The flagella sheath possesses a bilayered membrane, contains fatty acids characteristic of lipopolysaccharides, and is specifically labeled by a monoclonal antibody that reacts with a 29-kDa protein (39, 63).
Periplasmic fibers have been reported on H. felis, H. muridarum, H. bilis, H. trogontum, and "H. rappini" (80). They are located just below the outer mepmbrane in the periplasm and vary in number depending on the species. They have a different insertion point to flagella. Electron-dense bands can be seen, giving them a striated appearance (Fig. 1d). It has been suggested they are contractile proteins involved in motility (57).
Coccoid Forms
In common with many other spiral-shaped bacteria, members of the Helicobacter genus "ball up" and form coccoid cells as they age. After 3 to 4 days of bacterial culture coccoid cells dominate, and this is associated with a dramatic decrease in culturability as determined by CFU counts. Controversy exists as to whether these cells are viable, nonviable but dormant, or just dead. They have been implicated as possible reservoirs of bacteria exposed to harsh environmental conditions and involved in transmission of infection or as in vivo cells responsible for treatment failures. The ultrastructural changes that occur as H. pylori ages in in vitro culture have been well described and shown to be independent of the method used to induce coccoid formation (Fig. 3) (11, 55). Initially there is ingrowth in the periplasm on one side of the organism, with an accumulation of dense material that results in the formation of U-shaped cells. These "U" forms then convert to the coccoid form, with an increase in the protoplasmic cylinder and maintenance of the double membrane system. Analysis of the peptidoglycan during this conversion has shown dramatic modifications similar to that seen in Bacillus species during sporulation; however, the authors linked these changes to the growth phase rather than morphological changes (26).

Figure 3
Coccoid forms of H. pylori. (a) Initial ingrowth in the periplasmic space resulting in the formation of U-shaped cells. (b) Conversion to the coccoid form. Ultrathin section, bar = 0.5 μm.
Evidence supporting the concept that the coccoid forms are degenerate and not capable of growth comes from a number of studies showing that as the cells age, the levels of DNA and RNA and mRNA expression decrease with degradation of the nucleic acids, nonrandom fragmentation of the ribosomal RNA, and no evidence of a membrane potential necessary for processes such as oxidative phosphorylation (55, 70, 74). The protein profiles of coccoid forms changed from the original and have been linked to protein processing and degradation rather than de novo protein synthesis (55). However, there is conflicting evidence supporting the concept of a viable but dormant state as indicated by maintenance of cellular integrity and DNA synthesis in 3-month-old cultures. Electron-dense elements, identified by EELS as containing phosphorus, indicated metabolism could be maintained in the presence of the polyphosphates detected in the coccoid cells (18). Proteins such as CagA and UreC have been shown to decrease over time while others, e.g., 26-kDa and 16S rRNA, show no decrease over a 21-day period (87). Cell respiration also decreased over time but could still be detected in 40% of cells after 45 days of in vitro culture (23). A reduction in the detection of some but not all penicillin-binding proteins was seen during coccoid formation (29). Antigens specific to coccoid forms were also found to be expressed in vivo (11).
Opposing data regarding the ability of coccoid cells to infect a host and induce pathology exist, with the coccoid cells able to infect mice but not gnotobiotic piglets (22, 23, 101). The role of these coccoid forms in pathogenesis and transmission of infection needs clarification, with standardization of aspects such as the methods used to induce coccoid formation, determination of cell viability and resuscitation, and the length of time the cells have been exposed to unfavorable conditions. Proven nonviable cells need to be shown to be able to reinfect a host and be able to be cultured from the infected host before the coccoid forms can be considered truly a dormant form capable of reinfection.
In Vitro Effects of Antimicrobial Agents
The effects on the ultrastructure of H. pylori by a number of different agents have been described. A range of β-lactam antibiotics have been shown to induce the formation of spherical cells with greater detail showing the formation of cell wall "blebs," free membranous vesicles or tubular structures, and partial or complete disappearance of the external cell membrane (7, 29). These morphological changes have been attributed to the binding of one or more of the penicillin-binding proteins detected in H. pylori that result in inhibition of enzymes involved in peptidoglycan synthesis. The action of the only monolactam tested, aztreonam, was found to be different, with this antibiotic inducing filamentation in the H. pylori cells, and this was attributed to the fact that this antibiotic was the only one tested that bound preferentially to a single penicillin-binding protein thought to be essential for helical morphology (29).
Macrolide antibiotics resulted in clearing the central areas of cells, and this was associated with ribosomal clumping and impaired cross-wall development. No obvious structural changes were seen in the presence of metronidazole, ranitidine, or bismuth; however, with the latter, particulate bismuth complexes could be detected under the cell wall (7). Bismuth citrate was later shown to induce cell fragmentation and lysis as did ranitidine bismuth citrate, with the latter resulting in more severe disruption to the intracellular structure (105). Treatment with the proton pump inhibitor lansoprazole resulted in cell elongation and constriction and alterations to the cell surface (73). Many have postulated that these in vitro changes could be indicative of changes to H. pylori in human gastric mucosa during antibiotic treatment.
Ultrastructural aspects of infected tissue
The identification and characterization of ultrastructural features of H. pylori-infected tissue provide valuable insights into the pathogenesis of Helicobacter infection. However, the use of these characteristics to assess clinical outcomes of the infection is limited. This survey summarizes ultrastructural features representing the interaction of H. pylori virulence factors with defensive mechanisms of the host.
H. pylori and the gastric mucosal barrier
In the upper gastrointestinal tract, the gastric mucosal barrier represents the first line of defense against aggressive factors. Bacteria normally become trapped in the mucus and are excreted in feces (100). However, urease expression and motility permit H. pylori to survive transiently in an acid environment and to colonize persistently the mucous layer.
Gastric mucus
H. pylori is usually located within the thick mucous layer in close proximity to gastric epithelial cells (78). A weakening of the mucous barrier by H. pylori, leading in some cases to its collapse, has been proposed as H. pylori possesses a gene that is almost identical to a mucinase gene of Vibrio cholerae (75, 76, 91). Such mucinase activity may be responsible for the dissolution of the net-like structure of the mucus and the variously sized cave-like clear areas surrounding H. pylori (Fig. 4) as observed in vivo with electron microscopic techniques (14, 15). However, studies in vitro suggest that the loss of gel structure might also arise from high local pH generated by the urease activity of H. pylori rather than by mucolytic activity (2). Furthermore, H. pylori can inhibit the secretory response of mucous cells in vitro, indicating a potential deleterious effect on the quantity of this primary defense mechanism of the gastric mucosa (69).
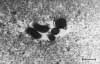
Figure 4
Ultrathin section of H. pylori colonization of gastric mucus. Bar, 1 μm.
Gastric hydrophobicity
A feature of the gastric mucosa that has been implicated with its barrier property is that of hydrophobicity (61). This surfactant is an essential component of the mucosal barrier and is due to an adsorbed monolayer or multilayer of surface-active phospholipids secreted by gastric mucous cells (Fig. 5) (46). This nonwettable barrier property has been documented to be present in a number of animal species by the use of specific electron microscopic methods (53).

Figure 5
Gastric surfactant of human antral mucosa. Specific jodoplatinate staining of phospholipid-rich structures. Ultrathin section; bar marker represents 0.5 μm. Mg, mucous granules; Lu, lumen.
Ultrastructural studies as well as contact angle measurements in humans indicate that H. pylori may act as an aggressive agent by destroying this gastric surfactant and its hydrophobic property (45, 67, 94). On the basis of animal studies in mice, Lichtenberger et al. conclude that H. felis infection induces an early transformation of the stomach from an hydrophobic to an acid-sensible hydrophilic state that may trigger the subsequent development of gastritis (60). This H. felis-induced alteration appears to be an early event, occurring during the first 2 weeks of the infection. Furthermore, the results indicate that the observed reduction in mucosal surface hydrophobicity and phospholipid concentration observed in human subjects infected with H. pylori also occurs in the H. felis-infected mouse. Enzymes that hydrolyze phospholipids by generating lysophosphatidylcholine or by decreasing the number of adsorbed surfactant molecules are likely to compromise this layer. The presence of phospholipase A2 (PLA2) and phospholipase C (PLC) activity has been demonstrated in H. pylori strains in vitro (56, 102).
Consequences of adhesion to epithelial cells
Generally, adhesion to mucosal surfaces is important for the full virulence of many bacterial pathogens in the human host. Binding promotes the delivery of bacterial toxins and often precedes penetration of target epithelial cells by invasive organisms. H. pylori recognizes specific epithelial cell receptors, and adherence seems to be an active process including gene transcription (90).
Pedestals
It was shown that H. pylori adhesion to gastric epithelial cells results in stunted microvilli and pedestal formation. The latter is associated with rearrangement of the cytoskeleton beneath the adherent bacterium and is termed attaching and effacing (90). The term attaching and effacing describes an intimate form of bacterial interaction with the plasma membrane of epithelial cells resulting from destruction of the microvillus cytoskeleton. In addition, polymerized actin under regions of bacterial attachment accumulates into adhesion pedestals at the bacterium host interface (71). These findings are similar to that described for the enteropathogenic Escherichia coli (EPEC), and this has been associated with genes encoded within a pathogenicity island (59). Furthermore, like EPEC, H. pylori binding to epithelial cells induces tyrosine phosphorylation of proteins in the host cell adjacent to the site of bacterial adherence (90). However, the information on the morphological changes and cytoskeleton rearrangements induced by the bacterium is contradictory (32, 49, 92). There is still a need for careful ultrastructural studies on the effects of an attachment of H. pylori to the gastric epithelial cells and subsequent events triggered by it. Contradictory results may be due to different cell lines used in the studies and probably are due to differences between in vitro and in vivo studies.
Because of contradictory data it seems important to describe ultrastructural features of H. pylori infection in animal models and compare these findings with those in humans. Recently, the Sydney strain of H. pylori has been shown to induce strikingly similar ultrastructural characteristics in a standardized mouse model, that is, close attachment and the formation of structures equivalent to the "adhesion pedestals" seen in human infection with H. pylori (58). Such adhesion pedestals have also been seen in ferrets, resulting from attachment of the ferret helicobacter, H. mustelae, with the gastric mucosa (81).
The development of specialized attachment sites represents an important aspect of pathogenicity, and their presence and frequency should be related to the degree of gastritis. It seems likely that toxins released by the bacteria are responsible for the cell damage and exert a maximal effect when concentrated in areas such as the cell membrane or intercellular spaces (20, 44, 77).
Cytotoxic effects
Biopsies of H. pylori-infected human gastric epithelium exhibit cellular swelling, cytoplasmic vacuolation, and expansion of endosomal compartments. However, these alterations are found to a lesser degree in vivo than in in vitro experiments using cell lines (93). This may be due to the more intense interaction of isolated virulence factors with cell cultures in vitro compared to the more complex situation in vivo, where various factors may interact.
Cytotoxin-associated gene antigen (CagA)
One of the most intensely studied proteins of H. pylori has been CagA, yet its function has not been determined. The CagA protein may be delivered from attached bacteria into the host cytoplasm by an H. pylori-specific type IV secretion system encoded by the cag pathogenicity island (cag PAI), where it is phosphorylated on tyrosine residues (95). It may then be able to influence eukaryotic signal transduction pathways and cytoskeletal plasticity (8, 89). The cag PAI has biological significance and may provide an explanation for the observation that H. pylori that produces CagA is more interactive with the host than those that do not. However, these effects are not visible at the ultrastructural level and do not show characteristic manifestations.
Vacuolating cytotoxin A (VacA)
Epidemiological and experimental evidence has clearly demonstrated the association between VacA production and the occurrence of tissue damage (98). The best known VacA effect is its ability to induce cytoplasmatic vacuoles in various eukaryotic cell lines by altering intracellular membrane traffic at the late endosomal/prelysosomal level. It is suggested that expression of H. pylori virulence is triggered by contact with the host cell. As seen for other pathogenic bacteria, the effector molecules of virulence are actively secreted on the host cell surface or even translocated across the mammalian host cell membrane (38, 68).
Vacuoles appear as round structures of up to several micrometers in diameter, a size that can be reached only upon fusion of several smaller compartments (Fig. 6). The toxin could promote endosome-endosome fusion either directly or through stimulation of a fusogenic protein. However, it is known that ammonia is also able to cause cell vacuolation (84, 88). The specific mechanisms by which the VacA causes these effects are not well understood, but recent studies indicate that VacA interacts directly with a cytoplasmatic target molecule (83). It is well established that epithelial barrier function depends on proper function of the cell cytoskeleton, and the observation that VacA disrupts cytoskeleton and focal adhesions may provide insight into the underlying mechanisms of these alterations.

Figure 6
Intracytoplasmatic vacuoles (V) in gastric epithelial cells induced in vivo by H. pylori. Ultrathin section. Bar, 0.5 μm.
Cytoskeleton
The cytoskeleton consists of microfilaments, microtubules, and intermediate filaments, which have contractile properties and participate in various cellular functions. Several bacterial toxins, such as Clostridium botulinum toxin or toxin A from Staphylococcus aureus, are known to cause alterations in cytoskeletal architecture (1, 103). Findings by Pai et al. suggest that H. pylori VacA cytotoxin disrupts cytoskeletal architecture and focal adhesions, thereby interfering with cytoskeleton-dependent cell functions and with the transmission of signals related to cell spreading and growth (83). Recently it was shown that VacA is strongly involved in interaction with intracellular filaments like actin and also between intermediate filaments and late endosomal compartments (28, 90, 96, 99). Rac, which belongs to the Rho GTPase family, regulates activity of the VacA cytotoxin in the cytoplasm, thus controlling the organization of the cytoskeleton (47). For this process, activation of genes and synthesis of new proteins are necessary (28). The biological implications of these findings are wide ranging. However, morphological investigations are confined to light microscopic investigations, and ultrastructural studies are lacking.
Tight junctions
There are only two pathways by which molecules can cross the epithelium: the transcellular and the paracellular. Gastric epithelial cells are tightly joined to each other, forming a continuous barrier that selectively restricts the movement of substances between the external and internal compartments. The tight junction appears to be the rate-limiting barrier that restricts flow through the paracellular pathway. Any disruption or modification of the epithelial architecture can lead to a decrease of the integrity of this barrier function (5). Ultrastructurally, the tight junction has been well defined. It appears in thin section as a series of individual contacts between the plasma membranes of adjacent epithelial cells. These points of contact correspond in freeze-fracture preparations to a variably complex network of fibrils and strands lying within the plane of the plasma membrane (5). In contrast to what might be expected from the analysis of electron micrographs that suggest a more static arrangement, the intestinal tight junctions are highly dynamic areas, and their permeability can change in response to both external and intracellular stimuli.
It has been shown in early studies that H. pylori is consistently associated with intercellular junctions in vivo, and it is proposed that this is due to the presence of preferred metabolites or growth factors (14, 42). Ohkusa et al. investigated the intercellular junctions in human gastric mucosa of patients with gastric ulcers and found a discontinuity and decrease in numbers of tight junctional strands, and Noach et al. observed that bacteria were often found around intercellular junctions with abnormalities of the tight junction complexes (Fig. 7) (77, 79). In addition, Terres et al. showed that H. pylori disrupts the barrier function of a model epithelium by producing a rapid increase in the permeability of tight junctions and a dramatic decrease in the transepithelial electrical resistance (TER) (99). TER changes reflect variation in tight junction integrity as shown by electron microscopy (65).
In vitro studies raise the possibility that VacA may be involved in selectively increasing permeability to ions and to low-molecular-mass molecules (85, 86). A decrease in TER was observed, but this did not necessarily result from disruption of the intercellular junctions. No sign of acute cytotoxicity or of intracellular vacuolization was observed. Therefore, the increased passage across the epithelial layer must follow a transcellular pathway. It is suggested that bacterial adherence to the epithelial cells is necessary to induce this transcellular passage. However, observed alterations of the tight junctions in vivo may be mainly due to the action of mediators of inflammation released by inflammatory cells in the gastric mucosa in response to H. pylori colonization. Recently, it has been speculated that actin plays a role in tight junction permeability (36, 43). Several bacterial toxins appear to affect the junction barrier through disruption of actin and by distortion and accumulation of junctional fibrils, as observed in freeze-fracture images. However, the part played by VacA—if any—in this process is still unclear.
Intracellular H. pylori
H. pylori has been considered to be an extracellular colonizer, but there are data supporting an intracellular location of bacteria in vivo and in vitro (3, 4, 14, 35, 62). Whereas in histopathologic studies tissue invasion by H. pylori is seldom reported, with only small numbers of bacteria occasionally seen within the lamina propria, H. pylori has been identified in the cytoplasm of gastric epithelial cells (6, 66). In addition, H. pylori antigens have been found in the lamina propria of the gastric mucosa (6, 66). Although H. pylori is not normally thought of as an invasive microorganism, electron microscopic studies of infected gastric mucosae have shown both intact viable bacteria and degraded forms within host cells (6, 24, 107).
Bacteria have been found within the mucous vacuoles or within the lysosomes of epithelial cells. Bode et al. have described the infiltration of gastric epithelial cells by H. pylori in 10% of their patients with active duodenal ulceration (14). However, the invasion was seen only in metaplastic surface mucous cells of the duodenum. Internalization of H. pylori by epithelial cells would provide a ready source of H. pylori antigens and a sustained immune response.
These in vivo observations are consistent with in vitro experiments using cell cultures (62, 96, 104). Evans et al. report that H. pylori could enter into the cytoplasm of cultured Hep-2 cells (35). It was concluded that H. pylori was internalized either by receptor-mediated endocytosis or by a closely related pathway. Birkness et al. were also able to demonstrate H. pylori within coated pits and H. pylori that appeared to have been taken into the host cells by endocytosis (13). Once taken into the cell, H. pylori was seen within vacuoles in the cultured epithelial cells.
The relevance of the above observations and the fate of intracellular organisms are still unknown, although a study has shown a correlation between high bacterial density of colonization, intracellular bacteria, and severe epithelial damage (24). Furthermore, there are observations that H. mustelae is also found within the cytoplasm of epithelial cells in the ferret. These data on bacterial internalization are also in line with the clinical observation that therapics using antibiotics unable to enter eukaryotic cells, such as amoxicillin, are less effective in eradicating H. pylori despite high in vitro susceptibility of the organism (48).
H. pylori, H. felis, and "H. heilmannii" have also been described in inactive, nonsecreting parietal cell canaliculi and within parietal cells (24, 50, 97). However, the pathophysiological consequence of interaction of parietal cells with H. pylori is not known, although the bacterium releases various factors capable of inhibiting parietal cells (21). In addition, H. pylori is phagocytosed by mononuclear phagocytes and by granulocytes (Fig. 8). However, the internalized bacteria are not killed and the reasons for this host defense defect are unclear (3, 25). These data support the hypothesis that intracellular H. pylori represents a reservoir of organisms that contributes to bacterial persistence, host tissue damage, and probably treatment failure.

Figure 8
H. pylori within the cytoplasm of a neutrophil granulocyte. Ultrathin section. Nu, nucleus, arrows, H. pylori. Bar, 1 μm.
Conclusion
Morphological and ultrastructural studies have provided important data as to the nature of the H. pylori–host interactions. These have aided researchers' quest to understand the complex processes leading to disease. These studies are also essential to the interpretation of data derived from animal experimentation.
References
- 1.
- Aepfelbacher M., Essler M., Huber E., Suggai M., Weber P. C. Bacterial toxins block endothelial wound repair Evidence that Rho GTPase control cytoskeletal rearrangements in migrating endothelial cells. Arterioscler. Thromb. Vasc. Biol. 1997;17:1623–1629. [PubMed: 9327754]
- 2.
- Allen A., Newton J., Oliver L., Jordan N., Strugula V., Pearson J. P., Dettmar P. W. Mucus and Helicobacter pylori. J. Physiol. Pharmacol. 1997;48:297–305. [PubMed: 9376612]
- 3.
- Allen L. A. Intracellular niches for extracellular bacteria: lessons from Helicobacter pylori. J. Leukoc. Biol. 1999;66:753–756. [PubMed: 10577505]
- 4.
- Allen L. A., Schlesinger L. S., Kang B. Virulent strains of Helicobacter pylori demonstrate delayed phagocytosis and stimulate homotypic phagosome fusion in macrophages. J. Exp. Med. 2000;191:115–128. [PMC free article: PMC2195807] [PubMed: 10620610]
- 5.
- Anderson J. M., van Itallie C. M. Tight junctions and the molecular basis for regulation of paracellular permeability. Am. J. Physiol. 1995;32:G467–G475. [PubMed: 7485497]
- 6.
- Andersen L. P., Holck S. Possible evidence of invasiveness of Helicobacter pylori. Eur. J. Clin. Microbiol. Infect. Dis. 1990;9:135–138. [PubMed: 2318218]
- 7.
- Armstrong J. A., Wee S. H., Goodwin C. S., Wilson D. H. Response of Campylobacter pyloridis to antibiotics, bismuth and an acid-reducing agent in vitro—an ultrastructural study. J. Med. Microbiol. 1987;24:343–350. [PubMed: 3694664]
- 8.
- Asahi M., Azuma T., Ito S., Ito Y., Suto H., Nagai Y., Tsubokawa M., Tohyama Y., Maeda S., Omata M., Suzuki T., Sasakawa C. Helicobacter pylori CagA protein can be tyrosine phosphorylated in gastric epithelial cells. J. Exp. Med. 2000;191:593–602. [PMC free article: PMC2195829] [PubMed: 10684851]
- 9.
- Austin J. W., Doig P., Stewart M., Trust T. J. Macromolecular structure and aggregation states of Helicobacter pylori urease. J. Bacteriol. 1991;173:5663–5667. [PMC free article: PMC208295] [PubMed: 1885543]
- 10.
- Austin J. W., Doig P., Stewart M., Trust T. J. Structural comparison of urease and a GroEL analog from Helicobacter pylori. J. Bacteriol. 1992;174:7470–7473. [PMC free article: PMC207446] [PubMed: 1358875]
- 11.
- Benaissa M., Babin P., Quellard N., Pezennec L., Cenatiempo Y., Fauchere J. L. Changes in Helicobacter pylori ultrastructure and antigens during conversion from the bacillary to the coccoid form. Infect. Immun. 1996;64:2331–2335. [PMC free article: PMC174074] [PubMed: 8675345]
- 12.
- Bereswill S., Waidner U., Odenbreit S., Lichte F., Fassbinder F., Bode G., Kist M. Structural, functional and mutational analysis of the pfr gene encoding a ferritin from Helicobacter pylori. Microbiology. 1998;144:2505–2516. [PubMed: 9782498]
- 13.
- Birkness K. A., Gold B. D., White E. H., Bartlett J. H., Quinn F. D. In vitro models to study attachment and invasion of Helicobacter pylori. Ann. N. Y. Acad. Sci. 1996;797:293–295. [PubMed: 8993385]
- 14.
- Bode G., Malfertheiner P., Ditschuneit H. Pathogenetic implications of ultrastructural findings in Campylobacter pylori related gastroduodenal disease. Scand. J. Gastroenterol. 1988;23(Suppl. 142):25–39. [PubMed: 3166531]
- 15.
- Bode G., Malfertheiner P., Mader U., Stanescu A., Ditschuneit H. Fine structure of active and healed duodenal ulcer. Am. J. Gastroenterol. 1991;86:179–186. [PubMed: 1704181]
- 16.
- Bode G., Malfertheiner P., Nilius M., Lehnhardt G., Ditschuneit H. Ultrastructural localisation of urease in outer membrane and periplasm of Campylobacter pylori. J. Clin. Pathol. 1989;42:778–779. [PMC free article: PMC1142036] [PubMed: 2760239]
- 17.
- Bode G., Mauch F., Ditschuneit H., Malfertheiner P. Identification of structures containing polyphosphate in Helicobacter pylori. J. Gen. Microbiol. 1993;139:3029–3033. [PubMed: 8126429]
- 18.
- Bode G., Mauch F., Malfertheiner P. The coccoid forms of Helicobacter pylori—criteria for their viability. Epidemiol. Infect. 1993;111:483–490. [PMC free article: PMC2271265] [PubMed: 8270008]
- 19.
- Brock F. M., Murray R. G. The ultrastructure and ATPase nature of polar membrane in Campylobacter jejuni. Can. J. Microbiol. 1988;34:594–604. [PubMed: 2974756]
- 20.
- Caselli M., Aleotti A., Boldrini P., Ruina M., Alvisi V. Ultrastructural patterns of Helicobacter pylori. Gut. 1993;34:1507–1509. [PMC free article: PMC1374411] [PubMed: 8244133]
- 21.
- Cave D. R. Helicobacter pylori and its interaction with chief and parietal cells. Yale J. Biol. Med. 1996;69:91–98. [PMC free article: PMC2588978] [PubMed: 9041695]
- 22.
- Cellini L., Allocati N., Angelucci D., Iezzi T., Dicampli E., L, Marzio, Dainelli B. Coccoid Helicobacter pylori not culturable in vitro reverts in mice. Microbiol. Immunol. 1994;38:843–850. [PubMed: 7898382]
- 23.
- Cellini L., Robuffo I., Dicampli E., Dibartolomeo S., Taraborelli T., Dainelli B. Recovery of Helicobacter pylori ATCC43504 from a viable but not culturable state—regrowth or resuscitation. APMIS. 1998;106:571–579. [PubMed: 9674895]
- 24.
- Chen X. G., Correa P., Offerhaus J., Rodriguez E., Jamey F., Hoffmann E., Fox J., Hunter F., Diavolitsis S. Ultrastructure of the gastric mucosa harboring campylobacter-like organisms. Am. J. Clin. Pathol. 1986;86:575–582. [PubMed: 2430450]
- 25.
- Chmiela M., Czkwianiane E., Wadström T., Rudnicka W. Role of Helicobacter pylori surface structures in bacterial interaction with macrophages. Gut. 1997;40:20–24. [PMC free article: PMC1027002] [PubMed: 9155570]
- 26.
- Costa K., Bacher G., Allmaier G., Dominguez-Bello M. G., Engstrand L., Falk P., de Pedro M. A., Garcia-del Portillo F. The morphological transition of Helicobacter pylori cells from spiral to coccoid is preceded by a substantial modification of the cell wall. Microbiology. 1999;181:3710–3715. [PMC free article: PMC93848] [PubMed: 10368145]
- 27.
- Cover T. L., Hanson P. I., Heuser J. E. Acid-induced dissociation of VacA, the Helicobacter pylori vacuolating cytotoxin, reveals its pattern of assembly. J. Cell Biol. 1997;138:759–769. [PMC free article: PMC2138037] [PubMed: 9265644]
- 28.
- deBernard M., Moschioni M., Napolitani G., Rappuoli R., Montecucco C. The VacA toxin of Helicobacter pylori identifies a new intermediate filament-interacting protein. EMBO J. 2000;19:48–56. [PMC free article: PMC1171776] [PubMed: 10619843]
- 29.
- DeLoney C. R., Schiller N. L. Competition of various beta-lactam antibiotics for the major penicillin-binding proteins of Helicobacter pylori: antibacterial activity and effects on bacterial morphology. Antimicrob. Agents Chemother. 1999;43:2702–2709. [PMC free article: PMC89546] [PubMed: 10543750]
- 30.
- Depamphilis M. L., Adler J. Attachment of flagellar basal bodies to the cell envelope: specific attachment to the outer, lipopolysaccharide membrane and the cytoplasmic membrane. J. Bacteriol. 1971;105:396–407. [PMC free article: PMC248367] [PubMed: 4250610]
- 31.
- Doig P., Trust T. J. Identification of surface-exposed outer membrane antigens of Helicobacter pylori. Infect. Immun. 1994;62:4526–4533. [PMC free article: PMC303139] [PubMed: 7927718]
- 32.
- Dytoc M., Gold B., Louie M., Huesca M., Fedorko L., Crowe S., Lingwood C., Brunton J., Sherman P. Comparison of Helicobacter pylori and attaching-effacing Escherichia coli adhesion to eukaryotic cells. Infect. Immun. 1993;61:448–456. [PMC free article: PMC302749] [PubMed: 8380793]
- 33.
- Eaton K. A., Catrenich C. E., Makin K. M., Krakowka S. Virulence of coccoid and bacillary forms of Helicobacter pylori in gnotobiotic piglets. J. Infect. Dis. 1995;171:459–462. [PubMed: 7844390]
- 34.
- Eaton K. A., Morgan D. R., Krakowka S. Motility as a factor in the colonisation of gnotobiotic piglets by Helicobacter pylori. J. Med. Microbiol. 1992;37:123–127. [PubMed: 1629897]
- 35.
- Evans D. G., Evans D. J., Graham D. Y. Adherence and internalization of Helicobacter pylori by Hep-2 cells. Gastroenterology. 1992;102:1557–1567. [PubMed: 1568565]
- 36.
- Fasano A., Fiorentini C., Donelli G., Uzzau S., Kaper J. B., Margaretten K., Ding X., Guandalini S., Comstock L., Goldblum S. E. Zonula occludens toxin (ZOT) modulates tight junctions through protein kinase C-dependent actin reorganization in vitro. J. Clin. Invest. 1995;96:710–720. [PMC free article: PMC185254] [PubMed: 7635964]
- 37.
- Ferris F. G., Beveridge T. J., Marccau-Day M. L., Larson A. D. Structure and cell envelope associations of flagellar basal complexes of Vibrio cholera and Campylobacter fetus. Can. J. Microbiol. 1984;30:322–333. [PubMed: 6426766]
- 38.
- Galan J. E., Bliska J. B. Cross-talk between bacterial pathogens and their host cells. Annu. Rev. Cell Dev. Biol. 1996;12:221–255. [PubMed: 8970727]
- 39.
- Geis G., Suerbaum S., Forsthoff B., Leying H., Opferkuch W. Ultrastructure and biochemical studies of the flagellar sheath of Helicobacter pylori. J. Med. Microbiol. 1993;38:371–377. [PubMed: 8487294]
- 40.
- Goodwin C. S., McCulloch R. K., Armstrong J. A., Wee S. H. Unusual cellular fatty acids and distinctive ultrastructure in a new spiral bacterium (Campylobacter pyloridis) from the human gastric mucosa. J. Med. Microbiol. 1985;19:257–267. [PubMed: 3981612]
- 41.
- Hawtin P. R., Stacey A. R., Newell D. G. Investigation of the structure and localization of the urease of Helicobacter pylori using monoclonal antibodies. J. Gen. Microbiol. 1990;136:1995–2000. [PubMed: 2269872]
- 42.
- Hazell S. L., Lee A., Brady L., Hennessy W. Campylobacter pyloridis and gastritis: association with intercellular spaces and adaption to an environment of mucus as important factors in colonization of the gastric epithelium. J. Infect. Dis. 1986;153:658–663. [PubMed: 3950447]
- 43.
- Hecht G., Pothoulakis G., LaMont J. T., Madara J. L. Clostridium difficile toxin a perturbs cytoskeletal structure and tight junction permeability of cultured human intestinal epithelial monolayers. J. Clin. Invest. 1988;82:1516–1524. [PMC free article: PMC442717] [PubMed: 3141478]
- 44.
- Hessey S. J., Spencer J., Wyatt J. I., Sobala G., Rathbone B. J., Axon A. T. R., Dixon M. F. Bacterial adhesion and disease activity in Helicobacter pylori associated chronic gastritis. Gut. 1990;31:134–138. [PMC free article: PMC1378366] [PubMed: 2311970]
- 45.
- Hills B. A. Gastric mucosal barrier: evidence for Helicobacter pylori ingesting gastric surfactant and deriving protection from it. Gut. 1993;34:588–593. [PMC free article: PMC1374172] [PubMed: 8504956]
- 46.
- Hills B. A., Butler B. D., Lichtenberger L. M. Gastric mucosal barrier: hydrophobic lining to the lumen of the stomach. Am. J. Physiol. 1983;244:G561–G568. [PubMed: 6846549]
- 47.
- Hotchin N. A., Cover T. L., Akhtar N. Cell vacuolation induced by the VacA cytotoxin of Helicobacter pylori is regulated by the Rac1 GTPase. J. Biol. Chem. 2000;275:14009–14012. [PubMed: 10747859]
- 48.
- Hulten K., Cars O., Hjelm E., Engstrand L. Invitro activity of azitromycin against intracellular Helicobacter pylori. J. Antimicrob. Chemother. 1996;37:493–499. [PubMed: 9182105]
- 49.
- Ismaili A., Philpott D. J., Dytoc M. T., Sherman P. M. Signal transduction responses following adhesion of verocytotoxin-producing Escherichia coli. Infect. Immun. 1995;63:3316–3326. [PMC free article: PMC173457] [PubMed: 7543880]
- 50.
- Jiang S. J., Liu W. Z., Zhang D. Z., Shi Y., Xiao S. D., Zhang Z. H., Lu D. Y. Campylobacter-like organisms in chronic gastritis, peptic ulcer, and gastric carcinoma. Scand. J. Gastroenterol. 1987;22:553–558. [PubMed: 3629180]
- 51.
- Jones D. M., Curry A., Fox A. J. An ultrastructural study of the gastric campylobacter-like organism "Campylobacter pyloridis. J. Gen. Microbiol. 1985;131:2335–2341. [PubMed: 4067580]
- 52.
- Josenhans C., Labigne A., Suerbaum S. Comparative ultrastructural and functional studies of Helicobacter pylori and Helicobacter mustelae flagellin mutants: both flagellin subunits, FlaA and FlaB, are necessary for full motility in Helicobacter species. J. Bacteriol. 1995;177:3010–3020. [PMC free article: PMC176987] [PubMed: 7768796]
- 53.
- Kao Y.-C. J., Lichtenberger L. M. A method to preserve extracellular surfactant-like phospholipids on the luminal surface of rodent gastric mucosa. J. Histochem. Cytochem. 1990;38:427–431. [PubMed: 1689341]
- 54.
- Kostrzynska M., Betts J. D., Austin J. W., Trust T. J. Identification, characterization, and spatial localization of two flagellin species in Helicobacter pylori flagella. J. Bacteriol. 1991;173:937–946. [PMC free article: PMC207209] [PubMed: 1704004]
- 55.
- Kusters J. G., Gerrits M. M., Vanstrijp J., Vandenbrouckegrauls C. Coccoid forms of Helicobacter pylori are the morphologic manifestation of cell death. Infect. Immun. 1997;65:3672–3679. [PMC free article: PMC175523] [PubMed: 9284136]
- 56.
- Langton S. R., Cesareo S. D. Helicobacter pylori associated phospholipase A2 activity: a factor in peptic ulcer production? J. Clin. Pathol. 1992;45:221–224. [PMC free article: PMC495476] [PubMed: 1556229]
- 57.
- Lee, A., and J. L. O'Rourke. 1993. Ultrastructure of Helicobacter organisms and possible revelance for pathogenesis, p. 15–35. In C. S. Goodwin and B. W. Worsley (ed.), Helicobacter pylori: Biology and Clinical Practice. CRC Press, Boca Raton, Fla.
- 58.
- Lee A., O'Rourke J., de Ungria M. C., Robertson B., Daskalopoulos G., Dixon M. F. A standardized mouse model of Helicobacter pylori infection: introducing the Sydney strain. Gastroenterology. 1997;112:1386–1397. [PubMed: 9098027]
- 59.
- Lee C. A. Pathogenicity islands and the evolution of bacterial pathogens. Infect. Agents Dis. 1996;5:1–7. [PubMed: 8789594]
- 60.
- Lichtenberger L. M., Dial E. J., Ottlecz A., Romero J. J., Lechago J., Fox J. G. Attenuation of hydrophobic phospholipid barrier is an early event in Helicobacter felis-induced gastritis in mice. Dig. Dis. Sci. 1999;44:108–115. [PubMed: 9952231]
- 61.
- Lichtenberger L. M., Graziani L. A., Dial E. J., Butler B. D., Hills B. A. Role of surface-active phospholipids in gastric cytoprotection. Science. 1983;219:1307–1309. [PubMed: 6828859]
- 62.
- Löfman C., Rigo R., Block M., Hulten K., Enroth H., Engstrand L. Bacterium-host interactions monitored by time-lapse photography. Nat. Med. 1997;8:939–931. [PubMed: 9256289]
- 63.
- Luke C. J., Penn C. W. Identification of a 29 kDa flagellar sheath protein in Helicobacter pylori using a murine monoclonal antibody. Microbiology (U.K.). 1995;141:597–604. [PubMed: 7711897]
- 64.
- Lupetti P., Heuser J. E., Manetti R., Massari P., Lanzavecchia S., Bellon P. L., Dallai R., Rappuoli R., Telford J. L. Oligomeric and subunit structure of the Helicobacter pylori vacuolating cytotoxin. J. Cell. Biol. 1996;133:801–807. [PMC free article: PMC2120837] [PubMed: 8666665]
- 65.
- Madara J., Pappenheimer J. Structural basis for physiological regulation of paracellular pathways in intestinal epithelia. J. Membr. Biol. 1987;100:149–164. [PubMed: 3430571]
- 66.
- Mai U. E. H., Perez-Perez G. I., Allen J. B., Wahl S. M., Blaser M. J., Smith P. D. Surface proteins from Helicobacter pylori exhibit chemotactic activity for human leukocytes and are present in gastric mucosa. J. Exp. Med. 1992;175:517–525. [PMC free article: PMC2119134] [PubMed: 1732414]
- 67.
- Mauch F., Bode G., Ditschuneit H., Malfertheiner P. Demonstration of a phospholipid-rich zone in the human gastric epithelium damaged by Helicobacter pylori. Gastroenterology. 1993;105:1698–1704. [PubMed: 8253346]
- 68.
- Mecsas J. J., Strauss E. J. Molecular mechanisms of bacterial virulence: type III secretion and pathogenicity islands. Emerg. Infect. Dis. 1996;2:270–288. [PMC free article: PMC2639918] [PubMed: 8969244]
- 69.
- Micots I., Augeron C., Laboisse C. L., Muzeau F., Megraud F. Mucin exocytosis: a major target for Helicobacter pylori. J. Clin. Pathol. 1993;46:241–245. [PMC free article: PMC501178] [PubMed: 8463418]
- 70.
- Monstein H. J., Tiveljung A., Jonasson J. Nonrandom fragmentation of ribosomal RNA in Helicobacter pylori during conversion to the coccoid form. FEMS Immunol. Med. Microbiol. 1998;22:217–224. [PubMed: 9848682]
- 71.
- Moon H. W., Whipp S. C., Argenzio R. A., Levine M. M., Giannella R. A. Attaching and effacing activities of rabbit and human enteropathogenic Escherichia coli in pig and rabbit intestines. Infect. Immun. 1983;41:1340–1351. [PMC free article: PMC264644] [PubMed: 6350186]
- 72.
- Murray R. G., Birch-Andersen A. Specialized structure in the region of the flagella tuft in Spirillum serpens. Can. J. Microbiol. 1963;9:393–401.
- 73.
- Nakao M., Tada M., Tsuchimori K., Uekata M. Antibacterial properties of lansoprazole alone and in combination with antimicrobial agents against Helicobacter pylori. Eur. J. Clin. Microbiol. Infect. Dis. 1995;14:391–399. [PubMed: 7556227]
- 74.
- Narikawa S., Kawai S., Aoshima H., Kawamata O., Kawaguchi R., Hikiji K., Kato M., Iino S., Mizushima Y. Comparison of the nucleic acids of helical and coccoid forms of Helicobacter pylori. Clin. Diagn. Lab. Immunol. 1997;4:285–290. [PMC free article: PMC170520] [PubMed: 9144365]
- 75.
- Newton J. L., Jordan N., Oliver L., Strugula V., Pearson J., James O. F. W., Allen A. Helicobacter pylori in vivo causes structural changes in the adherent gastric mucus layer but barrier thickness is not compromised. Gut. 1998;43:470–475. [PMC free article: PMC1727262] [PubMed: 9824571]
- 76.
- Newton J. L., Jordan N., Pearson J., Williams G. V., Allen A., James O. F. The adherent gastric antral and duodenal mucus gel layer thins with advancing age in subjects infected with Helicobacter pylori. Gerontology. 2000;46:153–157. [PubMed: 10754373]
- 77.
- Noach L. A., Rolf T. M., Tytgat G. N. J. Electron microscopic study of association between Helicobacter pylori and gastric and duodenal mucosa. J. Clin. Pathol. 1994;47:699–704. [PMC free article: PMC502139] [PubMed: 7962619]
- 78.
- Ogata M., Araki K., Ogata T. An electron microscopic study of Helicobacter pylori in the surface mucous gel layer. Histol. Histopathol. 1998;13:347–358. [PubMed: 9589892]
- 79.
- Ohkusa T., Yamamono M., Kataoka K., Kyoi T., Ueda F., Fujimoto H., Sasabe M., Tamura Y., Hosoi H., Tokoi S., Sasaki N., Saito Y. Electron microscopic study of intercellular junctions in human gastric mucosa with special references to their relationship to gastric ulcer. Gut. 1993;34:86–89. [PMC free article: PMC1374106] [PubMed: 8432458]
- 80.
- On, S. L., A. Lee, J. L. O'Rourke, F. E. Dewhirst, B. J. Paster, J. G. Fox, and P. A. R. Vandamme. 2000. Genus Helicobacter. In G. M. Garrity and D. J. Brenner (ed.), Bergey's Manual of Systematic Bacteriology, 2nd ed., vol. 2. Bergeys Manual Trust, New York, N.Y.
- 81.
- O'Rourke J. L., Lee A., Fox J. G. An ultrastructural study of Helicobacter mustelae and evidence of a specific association with gastric mucosa. J. Med. Microbiol. 1992;36:420–427. [PubMed: 1613782]
- 82.
- O'Toole P. W., Kostrzynska M., Trust T. J. Non-motile mutants of Helicobacter pylori and Helicobacter mustelae defective in flagellar hook production. Mol. Microbiol. 1994;14:691–703. [PubMed: 7891557]
- 83.
- Pai R., Cover T. L., Tarnawski A. Helicobacter pylori vacuolating cytotoxin (VacA) disorganizes the cytoskeletal architecture of gastric epithelial cells. Biochem. Biophys. Res. Comm. 1999;262:245–250. [PubMed: 10448099]
- 84.
- Papini E., deBernard M., Milia E., Bugnoli M., Zerial M., Rappuoli R., Montecucco C. Cellular vacuoles induced by Helicobacter pylori originate from the late endosomal compartments. Proc. Natl. Acad. Sci. USA. 1994;91:9720–9724. [PMC free article: PMC44888] [PubMed: 7937879]
- 85.
- Papini E., Satin B., Norais N., de Bernard M., Telford J. L., Rappuoli R., Montecucco C. Selective increase of the permeability of polarized epithelial cell monolayers by Helicobacter pylori vacuolating toxin. J. Clin. Invest. 1998;102:813–820. [PMC free article: PMC508944] [PubMed: 9710450]
- 86.
- Pelicic V., Reyrat J. M., Sartori L., Pagliaccia C., Rappuoli R., Telford J. L., Montecucco C., Papini E. Helicobacter pylori VacA cytotoxin associated with the bacteria increases epithelial permeability independently of its vacuolating activity. Microbiology. 1999;145:2043–2050. [PubMed: 10463170]
- 87.
- Ren Z., Pang G., Musicka M., Dunkley M., Batey R., Beagley K., Clancy R. Coccoid forms of Helicobacter pylori can be viable. Microbios. 1999;97:153–163. [PubMed: 10413871]
- 88.
- Ricci V., Sommi P., Fiocca R., Romano M., Solcia E., Ventura U. Helicobacter pylori vacuolating toxin accumulates within the endosomal-vacuolar compartment of cultured gastric cells and potentiates the vacuolating activity of ammonia. J. Pathol. 1997;183:453–459. [PubMed: 9496263]
- 89.
- Segal E. D., Cha J., Lo J., Falkow S., Tompkins L. S. Altered states: involvement of phosphorylated CagA in the induction of host cellular growth changes by Helicobacter pylori. Proc. Natl. Acad. Sci. USA. 1999;96:14559–14564. [PMC free article: PMC24475] [PubMed: 10588744]
- 90.
- Segal E. D., Falkow S., Tompkins L. S. Helicobacter pylori attachment to gastric cells induces cytoskeletal rearrangements and tyrosin phosphorylation of host cell proteins. Proc. Natl. Acad. Sci. USA. 1996;93:1259–1264. [PMC free article: PMC40067] [PubMed: 8577751]
- 91.
- Smith A. W., Chahal B., French G. I. The human gastric pathogen Helicobacter pylori has a gene encoding an enzyme first classified as a mucinase in Vibrio cholerae. Mol. Microbiol. 1994;13:153–160. [PubMed: 7984089]
- 92.
- Smoot D. T., Resau J. H., Naab T., Desbordes B. C., Gilliam T., Bull-Henry K., Curry S. B., Nidiry J., Sewehand J., Mills-Robertson K. Adherence of Helicobacter pylori to cultured human gastric epithelial cells. Infect. Immun. 1993;61:350–355. [PMC free article: PMC302729] [PubMed: 8418061]
- 93.
- Solcia E., Villani I., Luinetti O., Trespi E., Fiocca R. The mucosal response to Helicobacter pylori infection and its contribution to gastric pathology. Microecol. Ther. 1995;25:121–132.
- 94.
- Spychal R. T., Goggin P. M., Marrero J. M., Saverymuttu S. H., Yu C. W., Corbishley C. M., Maxwell J. D., Northfield T. C. Surface hydrophobicity of gastric mucosa in peptic ulcer disease: relationship to gastritis and Campylobacter pylori infection. Gastroenterology. 1990;98:1250–1254. [PubMed: 2323518]
- 95.
- Stein M., Rappuoli R., Covacci A. Tyrosine phosphorylation of the Helicobacter pylori CagA antigen after cag-driven host cell translocation. Proc. Natl. Acad. Sci. USA. 2000;97:1263–1268. [PMC free article: PMC15590] [PubMed: 10655519]
- 96.
- Su B., Johansson S., Fällman M., Patarroyo M., Granström M., Normark S. Signal transduction-mediated adherence and entry of Helicobacter pylori into cultured cells. Gastroenterology. 1999;117:595–604. [PubMed: 10464135]
- 97.
- Taniguchi Y., Kimura K., Satoh K., Yoshida Y., Kihira K., Takimoto T., Saifuku K., Ido K., Ookawara S., Mato M. Helicobacter pylori detected deep in gastric glands: an ultrastructural quantitative study. J. Clin. Gastroenterol. 1995;21:S169–S173. [PubMed: 8775013]
- 98.
- Telford J., Covacci A., Ghiara P., Montecucco C., Rappuoli R. Unravelling the pathogenic role of Helicobacter pylori in peptic ulcer: potential new therapies and vaccines. Trends Biotechnol. 1994;12:420–426. [PubMed: 7765388]
- 99.
- Terres A. M., Pajares J. M., Hopkins A. M., Murphy A., Moran A., Baird A. W., Kelleher D. Helicobacter pylori disrupts epithelial barrier function in a process inhibited by protein kinase C activators. Infect. Immun. 1998;66:2943–2950. [PMC free article: PMC108293] [PubMed: 9596771]
- 100.
- Wallace J. L., Granger D. N. The cellular and molecular basis of gastric mucosal defense. FASEB J. 1996;10:731–740. [PubMed: 8635690]
- 101.
- Wang X., Sturegard E., Rupar R., Nilsson H. O., Aleljung P. A., Carlen B., Willen R., Wadstrom T. Infection of BALB/c A mice by spiral and coccoid forms of Helicobacter pylori. J. Med. Microbiol. 1997;46:657–663. [PubMed: 9511813]
- 102.
- Weitkamp J. H., Perez-Perez G. I., Bode G., Malfertheiner P., Blaser M. J. Identification and characterization of Helicobacter pylori phospholipase C activity. Zentralbl. Bakteriol. 1993;280:11–27. [PubMed: 8280931]
- 103.
- Wiegers W., Just I., Muller H., Hellwig A., Traub P., Aktories K. Alteration of the cytoskeleton of mammalian cells cultured in vitro by Clostridium botulinum C2 toxin and C3 ADP-ribosyltransferase. Eur. J. Cell Biol. 1991;54:237–245. [PubMed: 1908779]
- 104.
- Wilkinson S. M., Uhl J. R., Kline B. C., Cockerill F. R. Assessment of invasion frequencies of cultured Hep-2 cells by clinical isolates of Helicobacter pylori using an acridine orange assay. J. Clin. Pathol. 1998;51:127–133. [PMC free article: PMC500507] [PubMed: 9602686]
- 105.
- Worku M. L., Sidebotham R. L., Karim Q. N. Effects of ranitidine bismuth citrate on Helicobacter pylori motility, morphology and survival. Aliment. Pharmacol. Ther. 1999;13:753–760. [PubMed: 10383504]
- 106.
- Worku M. L., Sidebotham R. L., Walker M. M., Keshavarz T., Karim Q. N. The relationship between Helicobacter pylori motility, morphology and phase of growth: implications for gastric colonization and pathology. Microbiology. 1999;145:2803–2811. [PubMed: 10537202]
- 107.
- Wyle F. A., Tarnawsky A., Schulman D., Dabros W. Evidence for gastric mucosal cell invasion by C. pylor: an ultrastructural study. J. Clin. Gastroenterol. 1990;12:S92–S98. [PubMed: 2212556]
- [The participation of some innate immunological defense components in children with chronic gastritis associated H. pylori infection in ultrastructure and function of the mast cells aspects].[Pol Merkur Lekarski. 2007][The participation of some innate immunological defense components in children with chronic gastritis associated H. pylori infection in ultrastructure and function of the mast cells aspects].Czkwianianc E, Janas B, Bartel H, Ryszard M, Durko A, Małecka-Panas E. Pol Merkur Lekarski. 2007 May; 22(131):346-9.
- Ultrastructure of the H. pylori microbe in individuals having macrocytosis and B12 deficiency.[Ultrastruct Pathol. 2011]Ultrastructure of the H. pylori microbe in individuals having macrocytosis and B12 deficiency.Khubchandani SR, Kulkarni S, Teckchandani S, Chitale A. Ultrastruct Pathol. 2011 Oct; 35(5):204-8. Epub 2011 Aug 25.
- Insights into the ultrastructural morphology of novel Planctomycetes.[Antonie Van Leeuwenhoek. 2013]Insights into the ultrastructural morphology of novel Planctomycetes.Lage OM, Bondoso J, Lobo-da-Cunha A. Antonie Van Leeuwenhoek. 2013 Oct; 104(4):467-76. Epub 2013 Jul 16.
- Review Host-environment interactions: their impact on progression from gastric inflammation to carcinogenesis and on development of new approaches to prevent and treat gastric cancer.[Cancer Epidemiol Biomarkers Pr...]Review Host-environment interactions: their impact on progression from gastric inflammation to carcinogenesis and on development of new approaches to prevent and treat gastric cancer.Wu MS, Chen CJ, Lin JT. Cancer Epidemiol Biomarkers Prev. 2005 Aug; 14(8):1878-82.
- Review [The type IV secretion system encoded by the cag PAI of Helicobacter pylori].[Wei Sheng Wu Xue Bao. 2007]Review [The type IV secretion system encoded by the cag PAI of Helicobacter pylori].Cui LL, Shao SH. Wei Sheng Wu Xue Bao. 2007 Aug; 47(4):743-5.
- Morphology and Ultrastructure - Helicobacter pyloriMorphology and Ultrastructure - Helicobacter pylori
- Profile neighbors for GEO Profiles (Select 55690218) (200)GEO Profiles
- Chromosome neighbors for GEO Profiles (Select 55654376) (20)GEO Profiles
- Chromosome neighbors for GEO Profiles (Select 55660704) (20)GEO Profiles
- Timm8a1 translocase of inner mitochondrial membrane 8A1 [Mus musculus]Timm8a1 translocase of inner mitochondrial membrane 8A1 [Mus musculus]Gene ID:30058Gene
Your browsing activity is empty.
Activity recording is turned off.
See more...