NCBI Bookshelf. A service of the National Library of Medicine, National Institutes of Health.
Committee on Predictive-Toxicology Approaches for Military Assessments of Acute Exposures; Committee on Toxicology; Board on Environmental Studies and Toxicology; Board on Life Sciences; Division on Earth and Life Studies; The National Academies of Sciences, Engineering, and Medicine. Application of Modern Toxicology Approaches for Predicting Acute Toxicity for Chemical Defense. Washington (DC): National Academies Press (US); 2015 Sep 1.
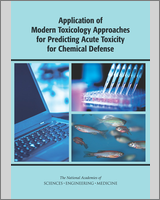
Application of Modern Toxicology Approaches for Predicting Acute Toxicity for Chemical Defense.
Show detailsAs discussed in Chapter 1, the committee was asked to consider modern approaches for predicting acute, debilitating chemical toxicity and to suggest an overall conceptual approach that uses emerging science to evaluate acute hazards to deployed military personnel. This chapter first discusses current and future needs for toxicity evaluations of chemical-warfare agents, recognizing the increasing number and types of chemicals that are potentially available to adversaries. It then describes the conceptual framework and strategy developed by the committee for systematically applying modern approaches to the prediction of acute toxicity. The overall approach, which is illustrated in Figures 2-1 and 2-2, consists of three components (relevant terms are defined in Box 2-1):
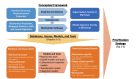
FIGURE 2-1
Conceptual framework and examples of databases, assays, models, and tools for predicting acute chemical toxicity.
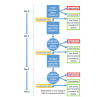
FIGURE 2-2
Prioritization strategy based on a tiered approach for using predictive-toxicology models and tools to evaluate agents for acute toxicity. The strategy can be applied to a single end point (such as lethality, neurotoxicity, and cytotoxicity) and to multiple (more...)
- A conceptual framework that links chemical structure, physicochemical properties, biochemical properties, and biological activity to acute toxicity.
- A suite of databases, assays, models, and tools that are based on modern in vitro, nonmammalian in vivo, and in silico approaches applicable to predicting acute toxicity.
- A tiered prioritization strategy for using databases, assays, models, and tools to predict acute toxicity in a manner that balances the need for accuracy and timeliness.
Later chapters in this report provide details of the types of databases, assays, models, and tools that are available for evaluating acute toxicity, their integration, and next steps that are needed to begin implementing the committee's framework and strategy.
ACUTE TOXICITY OF CLASSICAL CHEMICAL-WARFARE AGENTS
Historically, most chemical-warfare agents have belonged to the following chemical classes: nerve agents (such as sarin and soman), blister or vesicant agents (such as phosgene oxime and sulfur mustards), blood agents (such as cyanide), and pulmonary agents (such as chlorine and phosgene) (DHHS 2014). Those agents have been well studied, and a detailed mechanistic understanding that is based on human data is available for some. For example, the organophosphorus (OP) nerve agents are potent inhibitors of acetylcholinesterase and result in acute cholinergic effects that occur minutes or hours after exposure. Knowledge of their mechanisms of toxicity can be useful in the development of therapeutic countermeasures (Sharma et al. 2015) and in the development of in vitro tests. For example, in vitro methods have been developed for the evaluation of cholinesterase inhibition by nerve-gas agents (Worek et al. 2007). Data on some early, sensitive responses to such agents can support development of acute exposure limits for the general public. For example, a number of studies indicate that pupil constriction (miosis) is the most sensitive acute response to human exposure to OP nerve agents (such as sarin), and such end points have been used as part of the basis of acute exposure limits (NRC 2005).
In vivo testing approaches have been developed and applied to assess the toxicity of chemical-warfare agents. The vast majority of available toxicity information has come from traditional toxicity studies in which adverse biological responses were measured in laboratory animals that were exposed to high doses of a test agent. The acute-toxicity data are often used to provide estimates of the amount of an agent that would be required to kill 50% of a population of test animals, such as a lethal dose 50% (LD50) or a lethal concentration 50% (LC50). In addition, pharmacokinetic studies that were designed to identify species differences in chemical absorption, distribution, metabolism, and excretion (Tenberken et al. 2010; Benson et al. 2011a,b) and specialized pharmacokinetic models (such as ones that use a human or porcine skin flap) that were developed to evaluate absorption and toxicity of some chemical-warfare agents (Riviere et al. 1995; Monteiro-Riviere and Inman 1997; Vallet et al. 2008) have been undergoing incremental refinement since their inception. A limitation of the in vivo studies, however, is that they tend to be low-throughput, require consideration of species differences in response, and often provide little insight into a chemical's mechanism of action.
PREDICTING ACUTE TOXICITY OF POTENTIAL CHEMICAL-WARFARE AGENTS
Only a few chemicals have been formally classified as chemical-warfare agents. However, the list of chemicals that could potentially be used by an adversary against deployed US personnel is large and continues to grow as more chemicals enter the marketplace. Therefore, Department of Defense (DOD) efforts to evaluate potential chemical-warfare agents need to consider a wide array of chemicals beyond traditional chemical-warfare agents, including toxins of biological origin (such as trichothecenes, saxitoxin, and tetrodotoxin), industrial chemicals (such as ammonia), pesticides (such as sodium monofluoroacetate), and pharmaceutical agents (such as cocaine and amphetamine) (Holstege et al. 2007). The ability of an adversary to use those or other chemicals will depend on their or their precursors' availability and weaponizability and on other factors that were deemed beyond the scope of the committee's work but that might be important in deciding which agents to evaluate for acute toxicity.
To determine the best way to assess the growing list of registered chemical substances, the committee considered the adverse effects of highly toxic agents, including those of classical chemical-warfare agents, and identified the following organ systems to be of greatest importance for evaluating acute, debilitating hazards: cardiovascular, respiratory, hepatic, renal, skeletomuscular, immune, and nervous systems, including special senses (vision and hearing). Sufficient perturbation in those organ systems can lead to a progression in the severity of effects that can result in incapacitation or death of the whole organism.
Given ethical considerations, additional acute human-toxicity data are unlikely to be available except in cases of accidental release or deliberate attack for which exposure estimates are typically highly uncertain or unknown. And, available traditional toxicity-testing data provide little information about acute, debilitating toxicity. For example, information about chronic, reproductive, or developmental hazards—although important for chemical risk assessment in occupational or environmental settings—is of secondary concern in a military environment where acute, debilitating hazards are of immediate importance. As with other toxicity-testing programs, DOD recognizes that it would be prohibitively expensive and time-consuming to test all potential agents with traditional whole-animal toxicity-testing approaches even if such testing were limited to evaluations of acute toxicity. Moreover, traditional in vivo testing, particularly for acute toxicity, often does not provide information on the cellular or biological mechanisms of toxicity or in some cases even identify the target organ system.
Although some of the more modern, biological assay-based approaches have been used to elucidate mechanisms of action of many of the classical chemical-warfare agents described above, they have not been used to identify potential chemical-warfare agents. Nonetheless, the fact that some high-throughput screening data on chemical-warfare agents already exist suggests the feasibility of using such approaches to evaluate agents and provides important “reference” data with which results on other agents can be compared. The modern predictive approaches can also inform decisions as to whether additional mammalian in vivo testing of an agent is needed and might be able to provide information about the cellular and biological mechanistic events associated with acute toxicity and indicate whether additional testing should focus on a specific organ system or biological target.
A FRAMEWORK AND STRATEGY FOR PREDICTING ACUTE TOXICITY OF POTENTIAL CHEMICAL-WARFARE AGENTS
Conceptual Framework
A predictive-toxicology program to assess acute toxicity ideally will build on knowledge about the cellular targets and mechanisms of action that are related to acute human toxicity. Acute toxicity depends on fewer biological and chemical pathways than those envisioned by NRC (2007) for a general toxicity evaluation. It could be more straightforward, although still challenging, to predict the potential for acute toxicity than the potential for toxicity in the general public in a variety of organ systems, life stages, populations, and exposure timeframes. Specifically, clinical toxicologists have recognized several cellular or biological targets that are often associated with the acute lethal or debilitating effects of chemicals. Table 2-1 provides an overview of those cellular targets and relevant examples and lists some chemicals that affect the targets. It should be noted that there is not necessarily a one-to-one correspondence between mechanistic targets and organ-system targets because multiple mechanisms could affect a single organ system, a single mechanism could affect multiple organ systems, and debilitation or death could occur from multiorgan failure.
TABLE 2-1
Biological Processes and Cellular Targets Associated with Acute Toxicity in Humans or Laboratory Animals, .
The relatively detailed knowledge of the multiple mechanisms by which chemicals can cause acute toxicity supports the basic premise of predictive toxicology that whole-animal toxicity can be predicted on the basis of information on lower levels of complexity down to the level of chemical structure. That premise forms the basis of the conceptual framework developed by the committee, illustrated in Figure 2-1. Specifically, it is hypothesized that chemical structure, physicochemical properties, biochemical properties, or biological activity in isolated cells and tissues or in nonmammalian organisms can predict acute mammalian toxicity. The predictions can arise through observations of empirical or statistical correlations or through knowledge of the relevant mechanistic pathways, either of which could potentially be coupled with toxicokinetic information.
Databases, Assays, Models, and Tools
Evaluating the potential for acute toxicity by using the conceptual framework of predictive toxicology requires a suite of databases, assays, models, and tools to cover the relevant physical, chemical, biological, and toxicological space. In general, “input” information on chemical structure, physicochemical properties, biochemical properties, and biological activity that is used to make predictions will be obtained from relevant databases or assays. Chemical-structure data might range from chemical-grouping data (for example, reaction chemistry domains, such as Michael acceptors) to quantitative descriptors of chemical structure (for example, topological descriptors and semiempirical quantum chemical descriptors). Physicochemical-property data include quantities measured in physical or chemical assays, such as boiling point, pH, pKa, and KOW.1 Biochemical measures are usually measures of specific molecular interactions (such as DNA binding and receptor activation) with biological molecules, such as nucleic acids, proteins (including enzymes and receptors), and lipids. Finally, biological activity might include both specific measures of function (such as acetylcholinesterase inhibition) and nonspecific measures of toxicity (such as cytotoxicity from in vitro assays and LC50 estimates obtained from assays that use Drosophila). Databases and assays for chemical structures and physicochemical properties are discussed in Chapter 3 and Appendix B, and assays for biochemical properties and biological activity in Chapter 4.
In this same context, the prediction “outputs” consist of estimates of end points related to acute toxicity. The end points might be related to particular mechanisms known to cause acute toxicity (see, for example, Table 2-1), end points related to specific organ system targets (noted above), or nonspecific end points, such as death and cytotoxicity. Data on those end points for chemicals of known toxicity (such as classical chemical-warfare agents) can serve as “training” and “test” data for building models or tools to predict the same end points for chemicals on which such data are lacking. Finally, the specific form of the outputs might be qualitative (such as active or inactive), semiquantitative (such as a ranking), or quantitative (such as a numerical estimate of dose). However, as discussed further below, quantitative estimates are likely to be of greatest use for military applications.
A variety of models and tools might be used to provide toxicity estimates. Models and tools might be qualitative (such as decision trees) or quantitative (such as statistical regression) and might include statistically based (or machine-learning–based) models, biologically based models, or a mixture of the two. No model or tool is universally applicable, so it is important that a model's or tool's domain of applicability is characterized in terms of the chemical space in which it is predictive and the relevant toxicological end points that are covered. Moreover, toxicokinetic models might need to be integrated into the predictions to address absorption, distribution, metabolism, and excretion relevant to acute toxicity. Finally, models and tools differ with respect to the uncertainty or confidence in their predicted outputs.
As described further in Chapters 3-5, there are many available databases, assays, models, and tools that could be used to predict acute toxicity. Because they vary in their required level of effort, their relevance to acute toxicity, their domain of applicability, the extent to which they address toxicokinetics, and the uncertainty or confidence in their predictions, the committee developed an overall strategy for using them to evaluate acute toxicity. The committee's strategy is described next.
Prioritization Strategy for Evaluating Acute Toxicity
Effective implementation of predictive models and tools depends on first identifying the ultimate (and acceptable) use of the predictive outputs. The committee's task states that DOD needs to understand “the relative threat of the increasingly long list of registered chemical substances, particularly in terms of potential acute hazard.” The committee interprets that statement to mean that the goal of the predictive-toxicology approach is to prioritize substances in the sense of identifying those of greater and less concern for acute toxicity. Three key issues must be considered in developing a strategy for prioritization: the need for quantitative measures of potency and their uncertainties, the need to minimize false negatives, and the need to screen a large number of chemicals rapidly.
The first key issue is that prioritization with respect to toxicity inherently requires a quantitative measure of potency and a characterization of uncertainty. Ideally, potency should be defined in absolute units, such as an acute oral LD50 in milligrams per kilogram per day. Relative potency measures might be informative if they include reference chemicals that have known toxicity and, in that case, could be converted to absolute potency measures if toxicokinetic information is also available to make any necessary adjustments. Qualitative outputs, such as binary categorizations of “active” or “inactive,” might be useful as an additional output to target testing for specific end points but are not useful by themselves. Furthermore, in the absence of human data, there will always be inaccuracies in predicting human toxicity, so it is important to characterize the uncertainty or confidence associated with any predicted potency value. Because a decision-maker might have defined tolerance for errors (such as for false negatives and false positives2), the degree of uncertainty or confidence in a prediction can influence the decision that is made about a particular substance. Therefore, an estimated confidence interval is essential to any prioritization strategy.
The topic of uncertainty leads to the second key issue: given that this task is meant to prevent death and debilitating injuries of US military personnel, it is expected that there will be a low tolerance for false negatives. A likely consequence of reducing the number of false negatives is that a higher percentage of chemicals will be retained for assessment with more accurate but more resource-intensive approaches. The overall time needed to complete the review for the whole chemical space would increase accordingly. However, the timeframe to complete an assessment of thousands of chemicals could be unacceptably long, and a chemical could be successfully weaponized in that timeframe before a decision has been made.
The timeframe raises the third key issue: the prioritization strategy needs to be able to screen chemicals in a manner that allows rapid identification of the ones that pose the greatest risk. A rapid-screening scenario could be acceptable if follow-up screening is conducted to ensure that all potential chemical threats are eventually identified. It is critical that such an approach incorporate a short timeline that progresses efficiently through a multitiered approach to allow timely reconsideration of chemicals that are not originally classified as posing the greatest risk. Lessons learned from the first round of screening could then be leveraged effectively in the reassessment and enable a more informed review and follow-up validation of the initial approach that can also be rapidly implemented. The risk of using this approach lies in a time lag that could result in weaponization of a chemical that was originally not deemed to pose a great threat.
The policy tradeoff of balancing a low tolerance for false negatives with a need to identify important hazards rapidly is beyond the scope of the committee's charge. However, as a general approach, the committee found that the policy tradeoff could be managed through a tiered prioritization approach as illustrated in Figure 2-2. Specifically, the committee's proposed prioritization strategy proceeds through a number of tiers that apply successively more predictive and resource-intensive approaches than the previous ones. At each tier, a chemical is placed into one of three general categories:
- (a)
High confidence of low toxicity. These chemicals would be deselected for further study and are considered to have a low relative acute toxicity. The requirement that the determination be made with high confidence addresses the low tolerance for false negatives.
- (b)
High confidence of high toxicity. These chemicals would be selected and considered to have a high relative acute toxicity. The requirement that the determination be made with high confidence focuses attention quickly on chemicals that might pose a high risk.
- (c)
Uncertain toxicity due to inadequate data. The remaining chemicals would be candidates for moving to the next tier of evaluation for acute toxicity.3 The uncertainties might stem from available predictions of high uncertainty or low confidence or from inadequate coverage of end points deemed important for evaluating acute toxicity. Depending on resource constraints, it might be reasonable to assess the chemicals by using additional factors unrelated to toxicity, such as weaponizability. Thus, some chemicals might be further deselected for further study because they pose a low threat owing to factors unrelated to toxicity (discussion of such factors is beyond the committee's charge). If additional evaluation of toxicity is determined to be needed, the chemical would be moved to the next tier of hazard evaluation to reduce uncertainty concerning the potential for acute, debilitating toxicity. Uncertainty might also be reduced through additional research into and development of approaches to improve acute-toxicity prediction, that is, by decreasing the number of chemicals in category (c) and increasing the ability to discriminate between categories (a) and (b).
The categorization can be based on a single end point (possibly based on multiple approaches) or on multiple end points. The committee notes that an end point could be a clinical outcome or a molecular initiating event (see Figure 3-1). If science advances in such a way that adverse-outcome pathways of interest to DOD are known, the strategy shown in Figure 2-2 could rely on nontesting and biological assay-based approaches that evaluate molecular initiating events or measurable key events in the pathways.
The committee broadly grouped the available approaches to predicting acute toxicity into four tiers, beginning with an initial chemical characterization (Tier 0), proceeding to nontesting approaches (Tier 1), then to biological assay-based approaches (Tier 2), which includes nonmammalian animal species, and ultimately to traditional whole-animal toxicity testing (Tier 3). The tiers are described further in Box 2-2.
A key step in each tier is integration and decision-making (described in Chapter 5). Even within a tier, such as nontesting approaches (Tier 1), there might be diverse outputs and predictions from different models or tools that need to be synthesized. For example, a simple integration approach could be in the form of a scorecard that counts “positive” and “negative” results from available nontesting approaches; a more sophisticated integration approach might aggregate different predictions. In addition, Tier 2 integration should consider the previous results of nontesting approaches with the newly generated biological assay data. Absorption, distribution, metabolism, and excretion (ADME) considerations can be integrated to provide relevant information, such as chemical bioavailability or distribution to target organs.
The committee envisions that a decision as to whether a chemical is categorized as having high toxicity, low toxicity, or inadequate data could be made for each end point that is relevant to acute toxicity (see examples in Figure 2-1). As discussed previously, such decisions would be based on quantitative toxicity estimates for each toxicity end point and associated levels of confidence or confidence intervals. Defining the specific “thresholds” for assigning a chemical to each category will require expert judgment on the part of DOD. However, reference chemicals with known high and low toxicities could help to inform those boundaries. Overall, chemicals would also be assigned to categories for multiple individual end points that reflect different types of acute toxicity although, as noted in Chapters 3 and 4, there are many gaps in coverage of end points related to acute toxicity at all tiers. Therefore, noting the gaps as part of the prioritization strategy provides guidance on how to target testing in later tiers. And, it is up to DOD to determine the extent of coverage of end points that is adequate for it to make sufficiently reliable decisions at each tier.
FINDINGS AND RECOMMENDATIONS
- Finding: There are multiple mechanisms by which chemicals can elicit acute, debilitating toxicity, and these mechanisms provide support for a predictive-toxicology conceptual framework that predicts system, tissue, or organism toxicity on the basis of chemical structure, physicochemical properties, biochemical properties, or biological activity in isolated cells, tissues, and lower organisms.
- Finding: Such a conceptual framework that includes databases, assays, models, and tools that are applicable to prediction of acute toxicity could be used to evaluate a large number of chemicals for acute-toxicity potential more rapidly than traditional, mammalian in vivo studies.
- Finding: In prioritizing chemicals in terms of their potential to cause acute toxicity, DOD will need to balance a relatively low tolerance for false negatives with a need to evaluate a large number of chemicals rapidly. Regardless of how DOD decides to balance those objectives, they can be managed through a tiered prioritization strategy that applies successively more predictive and resource-intensive approaches as needed.
- Recommendation: The committee recommends a prioritization strategy that broadly groups approaches to prediction of acute toxicity into four tiers, beginning with an initial chemical characterization (Tier 0), moving to nontesting approaches (Tier 1), then to biological assay-based approaches (Tier 2), and finally to traditional mammalian in vivo testing (Tier 3). Progression through the tiers will require intermediate integration steps that consider the diverse data within a tier and among tiers. The prioritization strategy can be applied to single or multiple end points.
- Recommendation: As part of the prioritization strategy, the committee recommends placing chemicals into one of three general categories at each tier: “high confidence of high toxicity,” “high confidence of low toxicity,” and “inadequate data to evaluate toxicity confidently.” Chemicals placed in the last category, “inadequate data,” are moved to the next tier for additional, more resource-intensive evaluation. Quantitative estimates of how potent the chemicals might be and of the confidence or uncertainty in each estimate will be needed to place chemicals into categories. DOD will need to use expert judgment to define specifically how chemicals are to be assigned to the different categories.
REFERENCES
- Adams DJ, Boskovic ZV, Theriault JR, Wang AJ, Stern AM, Wagner BK, Shamji AF, Schreiber SL. Discovery of small-molecule enhancers of reactive oxygen species that are nontoxic or cause genotype-selective cell death. ACS Chem Biol. 2013;8(5):923–929. [PMC free article: PMC3658551] [PubMed: 23477340]
- Andacht TM, Pantazides BG, Crow BS, Fidder A, Noort D, Thomas JD, Blake TA, Johnson RC. An enhanced throughput method for quantification of sulfur mustard adducts to human serum albumin via isotope dilution tandem mass spectrometry. J. Anal. Toxicol. 2014;38(1):8–15. [PMC free article: PMC4539155] [PubMed: 24201816]
- Andersson S, Norman M, Olsson R, Smith R, Liu G, Nord J. High-precision, room temperature screening assay for inhibitors of microsomal prostaglandin E synthase-1. J. Biomol. Screen. 2012;17(10):1372–1378. [PubMed: 22895459]
- Attene-Ramos MS, Huang R, Sakamuru S, Witt KL, Beeson GC, Shou L, Schnellmann RG, Beeson CC, Tice RR, Austin CP, Xia M. Systematic study of mitochondrial toxicity of environmental chemicals using quantitative high throughput screening. Chem. Res. Toxicol. 2013;26(9):1323–1332. [PMC free article: PMC4154066] [PubMed: 23895456]
- Attene-Ramos MS, Huang R, Michael S, Witt KL, Richard A, Tice RR, Simeonov A, Austin CP, Xia M. Profiling of the Tox21 chemical collection for mitochondrial function to identify compounds that acutely decrease mitochondrial membrane potential. Environ. Health Perspect. 2015;123(1):49–56. [PMC free article: PMC4286281] [PubMed: 25302578]
- Bandi S, Viswanathan P, Gupta S. Evaluation of cytotoxicity and DNA damage response with analysis of intracellular ATM signaling pathways. Assay Drug Dev. Technol. 2014;12(5):272–281. [PMC free article: PMC4060777] [PubMed: 24927134]
- Benson JM, Tibbetts BM, Weber WM, Grotendorst GR. Uptake, tissue distribution, and excretion of 14C-sulfur mustard vapor following inhalation in F344 rats and cutaneous exposure in hairless guinea pigs. J. Toxicol. Environ. Health A. 2011a;74(13):875–885. [PubMed: 21598172]
- Benson JM, Gomez AP, Wolf ML, Tibbetts BM, March TH. The acute toxicity, tissue distribution, and histopathology of inhaled ricin in Sprague Dawley rats and BALB/c mice. Inhal. Toxicol. 2011b;23(5):247–256. [PubMed: 21473711]
- Büch TR, Schäfer EA, Demmel MT, Boekhoff I, Thiermann H, Gudermann T, Steinritz D, Schmidt A. Functional expression of the transient receptor potential channel TRPA1, a sensor for toxic lung inhalants, in pulmonary epithelial cells. Chem. Biol. Interact. 2013;206(3):462–471. [PubMed: 23994502]
- Chen Y, Guo C, Lim L, Cheong S, Zhang Q, Tang K, Reboud J. Compact microelectrode array system: Tool for in situ monitoring of drug effects on neurotransmitter release from neural cells. Anal Chem. 2008;80(4):1133–1140. [PubMed: 18271508]
- Cui HF, Ye JS, Chen Y, Chong SC, Sheu FS. Microelectrode array biochip: Tool for in vitro drug screening based on the detection of a drug effect on dopamine release from PC12 cells. Anal Chem. 2006;78(18):6347–6355. [PubMed: 16970308]
- deLemos AS, Foureau DM, Jacobs C, Ahrens W, Russo MW, Bonkovsky HL. Drug-induced liver injury with autoimmune features. Semin. Liver Dis. 2014;34(2):194–204. [PubMed: 24879983]
- DHHS (US Department of Health & Human Services). Chemical Warfare. Classes of Chemical Agents. Specialized Information System. 2014. [March 13, 2015]. http://sis
.nlm.nih.gov /enviro/chemicalwarfare.html#a1. - Dunlop J, Roncarati R, Jow B, Bothmann H, Lock T, Kowal D, Bowlby M, Terstappen GC. In vitro screening strategies for nicotinic receptor ligands. Biochem. Pharmacol. 2007;74(8):1172–1181. [PubMed: 17706607]
- Falk M, Hausmann M, Lukášová E, Biswas A, Hildenbrand G, Davídková M, Krasavin E, Kleibl Z, Falková I, Ježková L, Štefančíková L, Ševčík J, Hofer M, Bačíková A, Matula P, Boreyko A, Vachelová J, Michaelidesová A, Kozubek S. Determining Omics spatiotemporal dimensions using exciting new nanoscopy techniques to assess complex cell responses to DNA damage: Part A--radiomics. Crit. Rev. Eukaryot. Gene Expr. 2014;24(3):205–223. [PubMed: 25072147]
- Gutzkow KB, Langleite TM, Meier S, Graupner A, Collins AR, Brunborg G. High-throughput comet assay using 96 minigels. Mutagenesis. 2013;28(3):333–340. [PubMed: 23462850]
- Hill AJ, Jones NA, Smith I, Hill CL, Williams CM, Stephens GJ, Whalley BJ. Voltage-gated sodium (NaV) channel blockade by plant cannabinoids does not confer anticonvulsant effects per se. Neurosci. Lett. 2014;566:269–274. [PubMed: 24642454]
- Himmel HM. Drug-induced functional cardiotoxicity screening in stem cell-derived human and mouse cardiomyocytes: Effects of reference compounds. J. Pharmacol. Toxicol. Methods. 2013;68(1):97–111. [PubMed: 23702537]
- Holstege CP, Bechtel LK, Reilly TH, Wispelwey BP, Dobmeier SG. Unusual but potential agents of terrorists. Emerg. Med. Clin. North Am. 2007;25(2):549–566. [PubMed: 17482032]
- Huh D, Leslie DC, Matthews BD, Fraser JP, Jurek S, Hamilton GA, Thorneloe KS, McAlexander MA, Ingber DE. A human disease model of drug toxicity-induced pulmonary edema in a lung-on-a-chip microdevice. Sci. Transl. Med. 2012;4(159):159ra147. [PMC free article: PMC8265389] [PubMed: 23136042]
- Jellett JF, Marks LJ, Stewart JE, Dorey ML, Watson-Wright W, Lawrence JF. Paralytic shellfish poison (saxitoxin family) bioassays: Automated endpoint determination and standardization of the in vitro tissue culture bioassay, and comparison with the standard mouse bioassay. Toxicon. 1992;30(10):1143–1156. [PubMed: 1440621]
- Jensen KH, Rekling C. Development of a no-wash assay for mitochondrial membrane potential using the styryl dye DASPEI. J. Biomol. Screen. 2010;15(9):1071–1081. [PubMed: 20713988]
- Jin S, Sarkar KS, Jin YN, Liu Y, Kokel D, Van Ham TJ, Roberts LD, Gerszten RE, Macrae CA, Peterson RT. An in vivo zebrafish screen identifies organophosphate antidotes with diverse mechanisms of action. J. Biomol. Screen. 2013;18(1):108–115. [PMC free article: PMC4053346] [PubMed: 22960781]
- Jones JD, Kirsch HL, Wortmann RL, Pillinger MH. The causes of drug-induced muscle toxicity. Curr. Opin. Rheumatol. 2014;26(6):697–703. [PubMed: 25191992]
- Kelesidis T, Roberts CK, Huynh D, Martínez-Maza O, Currier JS, Reddy ST, Yang OO. A high throughput biochemical fluorometric method for measuring lipid peroxidation. HDL PLoS One. 2014;9(11):e111716. [PMC free article: PMC4219769] [PubMed: 25368900]
- Li Y, Feng X, Du W, Li Y, Liu BF. Ultrahigh-throughput approach for analyzing single-cell genomic damage with an agarose-based microfluidic comet array. Anal. Chem. 2013;85(8):4066–4073. [PubMed: 23477638]
- Liu S, Zhang Y, Moayeri M, Liu J, Crown D, Fattah RJ, Wein AN, Yu ZX, Finkel T, Leppla SH. Key tissue targets responsible for anthrax-toxin-induced lethality. Nature. 2013;501(7465):63–68. [PMC free article: PMC4080305] [PubMed: 23995686]
- Lopez-Izquierdo A, Warren M, Riedel M, Cho S, Lai S, Lux RL, Spitzer KW, Benjamin IJ, Tristani-Firouzi M, Jou CJ. A near-infrared fluorescent voltage-sensitive dye allows for moderate-throughput electrophysiological analyses of human induced pluripotent stem cell-derived cardiomyocytes. Am. J. Physiol. Heart Circ. Physiol. 2014;307(9):H1370–H1377. [PMC free article: PMC4217013] [PubMed: 25172899]
- Mioulane M, Foldes G, Ali NN, Schneider MD, Harding SE. Development of high content imaging methods for cell death detection in human pluripotent stem cell-derived cardiomyocytes. J. Cardiovasc. Transl. Res. 2012;5(5):593–604. [PMC free article: PMC3447146] [PubMed: 22896035]
- Monteiro-Riviere NA, Inman AO. Ultrastructural characterization of sulfur mustard-induced vesication in isolated perfused porcine skin. Microsc. Res. Tech. 1997;37(3):229–241. [PubMed: 9144635]
- Morrison KC, Hergenrother PJ. Whole cell microtubule analysis by flow cytometry. Anal. Biochem. 2012;420(1):26–32. [PubMed: 21893022]
- NRC (National Research Council). Impact of Revised Airborne Exposure Limits on Non-Stockpile Chemical Materiel Program Activities. Washington, DC: National Academies Press; 2005.
- NRC (National Research Council). Toxicity Testing in the 21st Century: A Vision and a Strategy. Washington, DC: National Academies Press; 2007.
- Pantazides BG, Crow BS, Garton JW, Quiñones-González JA, Blake TA, Thomas JD, Johnson RC. Simplified method for quantifying sulfur mustard adducts to blood proteins by Ultrahigh Pressure Liquid Chromatography-Isotope Dilution Tandem Mass Spectrometry. Chem. Res. Toxicol. 2015;28(2):256–261. [PMC free article: PMC4836402] [PubMed: 25622494]
- Pieperhoff S, Wilson KS, Baily J, de Mora K, Maqsood S, Vass S, Taylor J, Del-Pozo J, MacRae CA, Mullins JJ, Denvir MA. Heart on a plate: Histological and functional assessment of isolated adult zebrafish hearts maintained in culture. PLoS One. 2014;9(5):e96771. [PMC free article: PMC4019527] [PubMed: 24824845]
- Pointon A, Abi-Gerges N, Cross MJ, Sidaway JE. Phenotypic profiling of structural cardiotoxins in vitro reveals dependency on multiple mechanisms of toxicity. Toxicol. Sci. 2013;132(2):317–326. [PubMed: 23315586]
- Pointon A, Harmer AR, Dale IL, Abi-Gerges N, Bowes J, Pollard C, Garside H. Assessment of cardiomyocyte contraction in human-induced pluripotent stem cell-derived cardiomyocytes. Toxicol Sci. 2015;144(2):227–237. [PubMed: 25538221]
- Prasad RY, McGee JK, Killius MG, Suarez DA, Blackman CF, DeMarini DM, Simmons SO. Investigating oxidative stress and inflammatory responses elicited by silver nanoparticles using high-throughput reporter genes in HepG2 cells: effect of size, surface coating, and intracellular uptake. Toxicol. In Vitro. 2013;27(6):2013–2021. [PubMed: 23872425]
- Riviere JE, Brooks JD, Williams PL, Monteiro-Riviere NA. Toxicokinetics of topical sulfur mustard penetration, disposition, and vascular toxicity in isolated perfused porcine skin. Toxicol. Appl. Pharmacol. 1995;135(1):25–34. [PubMed: 7482537]
- Sakamuru S, Li X, Attene-Ramos MS, Huang R, Lu J, Shou L, Shen M, Tice RR, Austin CP, Xia M. Application of a homogenous membrane potential assay to assess mitochondrial function. Physiol. Genomics. 2012;44(9):495–503. [PMC free article: PMC3426425] [PubMed: 22433785]
- Scott CW, Zhang X, Abi-Gerges N, Lamore SD, Abassi YA, Peters MF. An impedance-based cellular assay using human iPSC-derived cardiomyocytes to quantify modulators of cardiac contractility. Toxicol Sci. 2014;142(2):331–338. [PubMed: 25237062]
- Sharma R, Gupta B, Singh N, Acharya JR, Musilek K, Kuca K, Ghosh KK. Development and structural modifications of cholinesterase reactivators against chemical warfare agents in last decade: A review. Mini Rev. Med. Chem. 2015;15(1):58–72. [PubMed: 25441834]
- Sirenko O, Cromwell EF, Crittenden C, Wignall JA, Wright FA, Rusyn I. Assessment of beating parameters in human induced pluripotent stem cells enables quantitative in vitro screening for cardiotoxicity. Toxicol. Appl. Pharmacol. 2013;273(3):500–507. [PMC free article: PMC3900303] [PubMed: 24095675]
- Sirenko O, Hesley J, Rusyn I, Cromwell EF. High-content assays for hepatotoxicity using induced pluripotent stem cell-derived cells. Assay Drug Dev. Technol. 2014a;12(1):43–54. [PMC free article: PMC3934660] [PubMed: 24229356]
- Sirenko O, Hesley J, Rusyn I, Cromwell EF. High-content high-throughput assays for characterizing the viability and morphology of human iPSC-derived neuronal cultures. Assay Drug Dev. Technol. 2014b;12(9-10):536–547. [PMC free article: PMC4270163] [PubMed: 25506803]
- Steinhoff RF, Ivarsson M, Habicher T, Villiger TK, Boertz J, Krismer J, Fagerer SR, Soos M, Morbidelli M, Pabst M, Zenobi R. High-throughput nucleoside phosphate monitoring in mammalian cell fed-batch cultivation using quantitative matrix-assisted laser desorption/ionization time-of-flight mass spectrometry. Biotechnol J. 2015;10(1):190–198. [PubMed: 25139677]
- Stenger B, Zehfuß F, Mückter H, Schmidt A, Balszuweit F, Schäfer E, Büch T, Gudermann T, Thiermann H, Steinritz D. Activation of the chemosensing transient receptor potential channel A1 (TRPA1) by alkylating agents. Arch. Toxicol. In press. [PubMed: 25395009]
- Sturm MB, Schramm VL. Detecting ricin: Sensitive luminescent assay for ricin A-chain ribosome depurination kinetics. Anal. Chem. 2009;81(8):2847–2853. [PMC free article: PMC2692192] [PubMed: 19364139]
- Suzuki OT, Frick A, Parks BB, Trask OJ Jr., Butz N, Steffy B, Chan E, Scoville DK, Healy E, Benton C, McQuaid PE, Thomas RS, Wiltshire T. A cellular genetics approach identifies gene-drug interactions and pinpoints drug toxicity pathway nodes. Front. Genet. 2014;5:272. [PMC free article: PMC4148776] [PubMed: 25221565]
- Tenberken O, Mikler J, Hill I, Weatherby K, Thiermann H, Worek F, Reiter G. Toxicokinetics of tabun enantiomers in anaesthetized swine after intravenous tabun administration. Toxicol. Lett. 2010;198(2):177–181. [PubMed: 20599598]
- Thoren TM, Thompson KS, Cardona PS, Chaturvedi AK, Canfield DV. In vitro absorption of atmospheric carbon monoxide and hydrogen cyanide in undisturbed pooled blood. J. Anal. Toxicol. 2013;37(4):203–207. [PubMed: 23482499]
- Thorn J. The inflammatory response in humans after inhalation of bacterial endotoxin: A review. Inflamm. Res. 2001;50(5):254–261. [PubMed: 11409488]
- Vale C, Alfonso A, Vieytes MR, Romarís XM, Arévalo F, Botana AM, Botana LM. In vitro and in vivo evaluation of paralytic shellfish poisoning toxin potency and the influence of the pH of extraction. Anal. Chem. 2008;80(5):1770–1776. [PubMed: 18232710]
- Vallet V, Cruz C, Licausi J, Bazire A, Lallement G, Boudry I. Percutaneous penetration and distribution of VX using in vitro pig or human excised skin validation of demeton-S-methyl as adequate simulant for VX skin permeation investigations. Toxicology. 2008;246(1):73–82. [PubMed: 18294748]
- van der Linden SC, von Bergh AR, van Vught-Lussenburg BM, Jonker LR, Teunis M, Krul CA, van der Burg B. Development of a panel of high-throughput reporter-gene assays to detect genotoxicity and oxidative stress. Mutat. Res. Genet. Toxicol. Environ. Mutagen. 2014;760:23–32. [PubMed: 24362253]
- Vangveravong S, McElveen E, Taylor M, Xu J, Tu Z, Luedtke RR, Mach RH. Synthesis and characterization of selective dopamine D2 receptor antagonists. Bioorg. Med. Chem. 2006;14(3):815–825. [PubMed: 16288878]
- Vongs A, Solly KJ, Kiss L, Macneil DJ, Rosenblum CI. A miniaturized homogenous assay of mitochondrial membrane potential. Assay Drug Dev. Technol. 2011;9(4):373–381. [PubMed: 21294696]
- Wasalathanthri DP, Malla S, Bist I, Tang CK, Faria RC, Rusling JF. High-throughput metabolic genotoxicity screening with a fluidic microwell chip and electrochemiluminescence. Lab. Chip. 2013;13(23):4554–4562. [PMC free article: PMC3901045] [PubMed: 24113555]
- Watson C, Ge J, Cohen J, Pyrgiotakis G, Engelward BP, Demokritou P. High-throughput screening platform for engineered nanoparticle-mediated genotoxicity using CometChip technology. ACS Nano. 2014;8(3):2118–2133. [PMC free article: PMC3971959] [PubMed: 24617523]
- Wijte D, Alblas MJ, Noort D, Langenberg JP, van Helden HP. Toxic effects following phosgene exposure of human epithelial lung cells in vitro using a CULTEX® system. Toxicol. In Vitro. 2011;25(8):2080–2087. [PubMed: 21945045]
- Wille T, Thiermann H, Worek F. Development of a high-throughput screening for nerve agent detoxifying materials using a fully-automated robot-assisted biological assay. Toxicol. In Vitro. 2010;24(3):1026–1031. [PubMed: 19961920]
- Wills LP, Beeson GC, Trager RE, Lindsey CC, Beeson CC, Peterson YK, Schnellmann RG. High-throughput respirometric assay identifies predictive toxicophore of mitochondrial injury. Toxicol. Appl. Pharmacol. 2013;272(2):490–502. [PMC free article: PMC3987703] [PubMed: 23811330]
- Worek F, Eyer P, Aurbek N, Szinicz L, Thiermann H. Recent advances in evaluation of oxime efficacy in nerve agent poisoning by in vitro analysis. Toxicol. Appl. Pharmacol. 2007;219(2-3):226–234. [PubMed: 17112559]
- Xiao J, Free RB, Barnaeva E, Conroy JL, Doyle T, Miller B, Bryant-Genevier M, Taylor MK, Hu X, Dulcey AE, Southall N, Ferrer M, Titus S, Zheng W, Sibley DR, Marugan JJ. Discovery, optimization, and characterization of novel D2 dopamine receptor selective antagonists. J. Med. Chem. 2014;57(8):3450–3463. [PMC free article: PMC4315423] [PubMed: 24666157]
- Yakushenko A, Kätelhön E, Wolfrum B. Parallel on-chip analysis of single vesicle neurotransmitter release. Anal. Chem. 2013;85(11):5483–5490. [PubMed: 23642073]
- Zielonka J, Cheng G, Zielonka M, Ganesh T, Sun A, Joseph J, Michalski R, O'Brien WJ, Lambeth JD, Kalyanaraman B. High-throughput assays for superoxide and hydrogen peroxide: Design of a screening workflow to identify inhibitors of NADPH oxidases. J. Biol. Chem. 2014;289(23):16176–16189. [PMC free article: PMC4047388] [PubMed: 24764302]
Footnotes
- 1
Kow is the octanol-water partition coefficient.
- 2
In this context, a “false negative” occurs when a chemical is identified as having low toxicity when it actually has high toxicity, and “false positive” occurs when a chemical is identified as having high toxicity when it actually has low toxicity.
- 3
Some chemicals in categories (a) and (b) might be carried to a higher tier for validation purposes.
- Conceptual Framework and Prioritization Strategy - Application of Modern Toxicol...Conceptual Framework and Prioritization Strategy - Application of Modern Toxicology Approaches for Predicting Acute Toxicity for Chemical Defense
Your browsing activity is empty.
Activity recording is turned off.
See more...