This is an open-access article distributed under the terms of the Creative Commons Attribution License, which permits unrestricted use, distribution, and reproduction in any medium, provided the original work is properly cited.
NCBI Bookshelf. A service of the National Library of Medicine, National Institutes of Health.
StemBook [Internet]. Cambridge (MA): Harvard Stem Cell Institute; 2008-. doi: 10.3824/stembook.1.5.1
The two definitive characteristics of stem cells, the capacity for proliferation without loss of potency and the ability to differentiate into specialized cell type(s), endow these cells with great promise for the development of cell replacement therapies for degenerative disease and injury. Furthermore, stem cells are increasingly being recognized as versatile systems for developmental biologists to study lineage specification and tissue organization.
Understanding the mechanisms that underlie stem cell self-renewal and differentiation is central to realizing this potential, and efforts have increasingly focused on elucidating the signals that regulate cell function in the tissues where they reside, i.e. the endogenous stem cell niche. The governing principle of this niche, first proposed 30 years ago, is that stem cell fate decisions are influenced by cells’ interactions with components of their microenvironment, and these cell extrinsic factors include soluble and immobilized factors, the extracellular matrix, and signals presented by neighboring cells. Thus, recreating or simulating this microenvironment may be critical for properly expanding and controlling the differentiation of stem cells outside the body, for both basic biological study and therapeutic translation.
In this chapter we review the biology of stem cell niches, focusing on the mechanisms and “strategies” by which various niche components support stem cell functions, including immobilization of signals to control activity and dosage, progressive specification of cell behavior by a series of sequential signals, complex signaling feedback from adjacent niche cells, and presentation of mechanical cues. We then illustrate how these mechanisms can be translated into design criteria for stem cell engineers to create biomimetic microenvironments that emulate the ability of native niches to precisely control cell function.
Introduction
Stem cells – defined by the capacities for self-renewal and for differentiation into multiple cell types – hold great promise for applications in regenerative medicine and as model systems for basic developmental biology studies. Tissue-specific adult stem cells have been isolated from a broad variety of adult tissues in many species. In addition to these multipotent stem cells, pluripotent embryonic stem cells that have the ability to generate all cells in an adult organism have been isolated from embryos (Martin, 1981; Thomson et al., 1998), and induced pluripotent stem cells (iPS) have been created from somatic cells via the forced overexpression of key factors (Takahashi et al., 2007; Takahashi and Yamanaka, 2006). Understanding how to preciously control the behavior of these cells is a key challenge for utilizing them in both therapeutic and scientific applications.
The hallmark properties of stem cell self-renewal and differentiation are governed by a complex set of extrinsic cues in collaboration with intrinsic gene regulatory machinery. The identification of numerous tissue-specific stem cells has driven recent investigations into whether there are intrinsic regulatory mechanisms shared by all self-renewing cells (Ivanova et al., 2002; Ramalho-Santos et al., 2002). However, gene expression profiling studies across many different types of stem cells have not been able to define a common, core set of genes responsible for “stemness” (Fortunel et al., 2003). This finding in part has led to a perceptible shift in focus from the role of intrinsic regulation to the function of cell extrinsic stimuli as determinants of cell fate. The conceptual framework for the latter efforts was initially proposed in 1978 by Schofield, who hypothesized that the soluble growth factors, extracellular matrix interactions, and cell-cell interactions that comprise the stem cell microenvironment play a role in supporting stem cell self-renewal (Schofield, 1978). Accordingly, the identification of extrinsic factors and their downstream, intracellular signaling pathway targets has led to much progress in understanding how to control the expansion and directed differentiation of stem cells.
In parallel to these basic efforts, engineered microenvironments have been increasingly successful in controlling stem cell fate by emulating the key regulatory signals from native stem cell niches. This chapter has the dual objectives of discussing principles of stem cell biology, focusing on the strategies and mechanisms by which niche components support stem cell functions, as well as illustrating key examples of engineered stem cell microenvironments that build from these principles. We present examples of how growth factors and morphogens, neighboring cells, and the extracellular matrix regulate the size and composition of the niche (see Figure 1). Since there are many more cell types in organisms than there are signaling factors, biology encodes information that specifies cell fate not only through the presence or absence of signals, but also via their combinations, localization, level, and timing. The examples of engineered microenvironments discussed below indicate how a detailed understanding of how these components regulate stem cells in native niches has led to the elucidation and development of key design criteria for synthetic niches that allow greater control over cell fate.
Growth factors and morphogens
Immobilization of signaling factors as a mechanism to control local concentration
Growth factors and morphogens can exert potent, long-range effects in stem cell microenvironments. Owing to their relative ease of study, soluble growth factors and their downstream signal transduction pathways are the best characterized determinants of stem cell fate and have been extensively used in ex vivo stem cell culture systems, as has been effectively discussed elsewhere (Lowry and Richter, 2007; Molofsky et al., 2004). We will instead discuss the application of growth factor immobilization, a ubiquitous theme in developmental biology, to engineered niches.
In vivo, numerous growth factors and morphogens are immobilized by binding to the extracellular matrix through specific heparin-binding domains or by direct binding to ECM molecules such as collagen as well as by fibronectin, or direct anchoring to cell membranes (Rider, 2006). Immobilization of growth factors in this manner can serve to increase local concentration of the protein by hindering diffusion and receptor-mediated endocytosis. For example, the morphogen Sonic hedgehog (Shh) is modified at its termini by lipids – cholesterol and palmitic acid – that link it to the cell membrane and thereby limit its mobility. Removing the lipids dilutes the factor to a lower concentration and thereby shrinks the range of its effective activity (Li et al., 2006; Saha and Schaffer, 2006). Accordingly, mimicking the natural immobilization of cytokines is one approach utilized by engineers to concentrate factors in proximity to the cell surface in a manner that effectively activates target signaling pathways, as well as reduces the levels of growth factor necessary to elicit a potent cellular response.
An early study exploring this design concept focused on epidermal growth factor (EGF; Kuhl and Griffith-Cima, 1996), which is effective in repair of damaged tissues, but is often difficult to deliver at a sufficiently high concentrations to mediate downstream signaling events since it does not contain a matrix-binding domain and rapidly undergoes receptor-mediated endocytosis (Reddy et al., 1996). Immobilization of EGF on a biomaterial surface in a manner that preserved its activity but precluded receptor-mediated downregulation was first explored as a way to achieve high local concentration and sustained signaling in primary rat hepatocytes (see Figure 2; Kuhl and Griffith-Cima, 1996). In a recent example involving human and porcine mesenchymal stem cells (MSCs), amine-targeting chemistry was used to tether EGF to the surface of poly(methyl methacrylate)-graft-poly(ethylene oxide) (PMMA-g-PEO) comb polymers (Fan et al., 2007). The tethered EGF led to sustained EGFR signaling and subsequent cellular responses including cell spreading and protection from apoptosis whereas saturating levels of soluble EGF did not (Fan et al., 2007).
Motivated by naturally-occurring heparin-binding domains, Sakiyama-Elbert and colleagues incorporated heparin into biomaterial scaffolds to allow for immobilization of basic fibroblast growth factor (bFGF), a growth factor with many important roles during development and in adult physiology (Sakiyama-Elbert and Hubbell, 2000). bFGF was released either passively by diffusion, or actively via heparinases secreted by neighboring cells, thereby allowing for a controlled release and presentation of signal not possible with soluble growth factor delivery. In a chick dorsal root ganglion model, immobilized bFGF led to greater neurite extension into the scaffold than for cells cultured on scaffolds with free bFGF (Sakiyama-Elbert and Hubbell, 2000), likely due in part to the finding that the local concentration in the scaffold was 500-fold greater for immobilized bFGF than for soluble FGF. The same delivery system has been used for differentiation of murine ESCs into mature neural cell types, including neurons and oligodendrocytes, indicating that biomaterials scaffolds functionalized with immobilized growth factors may be a potential strategy for generation of engineered tissue for treatment of spinal cord injury (Willerth, 2008).
Finally, in a recent study, polymer substrates functionalized with the signaling domain of Sonic hedgehog (Shh) supported enhanced osteogeneic differentiation of bone marrow-derived MSCs, as compared to cells cultured on the same surfaces with soluble Shh at the same concentration (Ho et al., 2007). This example further demonstrates that growth factor or morphogen immobilization serves as an effective means to achieve sustained activation of downstream signaling pathways.
Sequential factors to program stem cell differentiation
Decades of elegant studies in numerous model organisms have elucidated signals and molecular pathways that sequentially and progressively regulate the generation of terminally differentiated cell types during the process of organismal development (see Figure 2). One of the major accomplishments of embryonic stem cell biology thus far has been to apply this concept of a step-wise developmental program in vitro to recapitulate organ formation. In particular, generation of functional motor neurons and pancreatic cells are illustrative examples of how sequential soluble factors can be used to program stem cell differentiation.
Differentiation of motor neurons from primitive ectoderm requires progression through two intermediate stages, each guided by a distinct set of soluble factors. Ectodermal cells first acquire a rostral neural fate through signaling by BMP, FGF, and Wnt proteins (Munoz-Sanjuan and Brivanlou, 2002). The next transition is retinoic acid-mediated caudalization, followed by progression to terminally differentiated motor neurons in the presence of Shh (Briscoe and Ericson, 2001). In a landmark study, Jessell and colleagues were able to guide mouse ESCs down this pathway by applying these soluble factors in a step-wise manner that emulates natural neural development, resulting in the in vitro generation of motorneurons that can survive and engraft in vivo (Wichterle et al., 2002).
Analogous sequential exposure to soluble factors has enabled recapitulation of pancreatic development in vitro. The pancreas develops from the embryonic endoderm, first appearing as buds at two sites of the gut tube. Progression to the next stage of development, denoted by expression of pancreatic-specific transcription factors, depends on Shh-antagonists secreted by the embryonic mesoderm (Hebrok et al., 1998; Kim et al., 1997). Further growth and branching of the nascent pancreatic buds is mediated by FGF10 (Bhushan et al., 2001). This step is followed by endocrine cell specification, which is mediated by soluble signals from the surrounding endothelium that inhibit Notch signaling and subsequently initiate expression a cascade of transcription factors that lead to differentiation of insulin-producing cells (Gu et al., 2003; Lammert et al., 2001). Application of these soluble signals in sequence to undifferentiated embryonic stem cells in vitro resulted in the generation of functional insulin-producing cells (D’Amour et al., 2005; D’Amour et al., 2006), illustrating an important concept for directed differentiation of ESCs.
Factors secreted or presented from differentiated cells within the niche
Stem cells reside in complex and heterogeneous compartments throughout the body, and interactions with one another and with surrounding cells can modulate their fate decisions. Therefore, the specific balance of cell types within the niche is a regulator of stem cell behavior. In some regions of the adult brain, signaling feedback from differentiated cells inhibits the proliferation of progenitor cells in order to maintain the balance of cell types in the niche. Wu et al. identified a TGF-β family member that inhibited neurogenesis in the olfactory epithelium, and then showed that it was produced by differentiated neurons (Wu et al., 2003). This system demonstrates the concept that differentiated cells can express a soluble protein to exert negative feedback control over stem cell proliferation, thereby maintaining a balance of signals and cell types within the local microenvironment.
Similar paracrine signaling has been shown to regulate human embryonic stem cell (hESC) cultures. Even when not grown on embryonic fibroblast or other feeder cells, hESCs grow in heterogeneous colonies composed of undifferentiated cells and hESC-derived fibroblast-like cells that do not express ES cell markers (Stewart et al., 2006). Recent work suggests that the fibroblast-like cells form a part of the hESC microenvironment by secreting factors necessary for stem cell self-renewal (Bendall et al., 2007). Specifically, fibroblasts derived from hESCs were shown to secrete insulin-like growth factor (IGF) II, which interacts with the IGF receptor I expressed on the surface of undifferentiated hESCs.
A closely related parameter is overall cell density. As one example, the Notch signaling pathway mediates the process of lateral inhibition, where a cell presenting a Notch ligand stimulates Notch activity in adjacent cells, which, in addition to modulating the recipient cell’s fate, also downregulates the expression of Notch ligands on that cell. This signaling mechanism regulates key stages of neural development in both vertebrates and invertebrates. For example, specification of neuronal ganglion cells in the retina from a population of competent progenitors is mediated by Notch signaling such that inhibition of Notch leads to differentiation (Austin et al., 1995). A similar juxtracine signaling relationship is observed in in vitro neural stem cell cultures in which high cell density exerts an inhibitory effect on neuronal differentiation, which can be abrogated by the addition of antisense oligonucleotides directed against Notch (Bartlett et al., 1998).
One strategy to engineer paracrine and potentially juxtracrine regulation into a synthetic stem cell environment is to culture stem cells alongside more differentiated cell types. While these co-culture systems serve as the in vitro analog of native environments comprised of multiple cell types, they are often difficult to design and optimize, as well as to precisely control for basic investigation of paracrine signaling. Recently, tissue engineers have developed technologies that address some of the challenges of co-culture systems. For example, Hui and Bhatia used microfabricated, interdigitating silicon combs to control cell-cell interactions over time (Hui and Bhatia, 2007) (see Figure 3). In this system, cells are cultured on silicon combs whose spacing can be modulated over time. Micromechanical control of comb spacing allows for the modulation of cell-cell contacts and spacing to explore the roles of contact-mediated versus soluble signals among heterogeneous cell types. For example, dynamic regulation of the spacing between hepatocytes and stromal cells revealed that stromal contact is only needed at an early time interval to sustain hepatocellular function. Similarly, Wright et al. used a microstencil to pattern various cell types layer by layer over time (Wright et al., 2007). This system allows for the optimization of each layer for each specific cell type (e.g. adhesive substrate coating), as well as the addition of cells in the co-culture in a defined sequence. Each of these systems represents emerging technologies that are a step towards recreating and exploring the complex dynamic cell-cell interactions that occur in stem cell niches.
Another approach to regulating cell-cell contacts in synthetic stem cell niches has been to regulate overall cell density. Negative feedback mechanisms mediated by cell-cell contacts can serve to maintain the balance of cell types in some stem cell niches, and controlling cell density can thus be considered a simpler proxy to recreating complex cell-cell contacts. One example comes from embryonic stem cell culture. In order to begin to parse the complex cell-cell interactions in hESC culture, Zandstra and colleagues have studied the effect of local cellular microenvironments on intracellular signaling pathways (Peerani et al., 2007). Using high-resolution imaging, they showed that hESCs retain stem cell marker expression upon growth factor removal in regions of high local cell density within hESC colonies. Nuclear levels of signaling molecules such as phosphorylated Smad1, a component of the TGF-β pathway known to function in hESC self-renewal, were also found to be dependent on local cell density. Further analysis suggested that differentiated cells secrete Smad1 agonists, while undifferentiated cells secrete Smad1 antagonists. Moreover, using engineered surfaces with micropatterned cell adhesive features, they showed that controlling hESC colony size can be used to modulate the level of Smad signaling and maintenance of self-renewal. Their findings suggest that self-renewal requires a balance between TGF-β agonists and antagonists, and that this balance is determined by the subpopulations and densities of cells that comprise the local cell microenvironment.
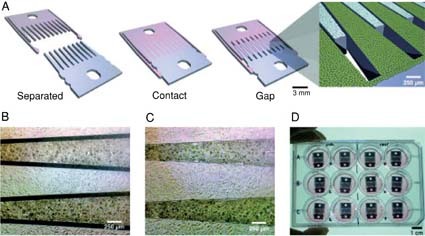
Figure 4Micromechanical substrates enable micrometer-resolution cell positioning.
(A) Microfabricated silicon parts can be fully separated (Left), locked together with comb fingers in contact (Center), or slightly separated (Right). (B and C) Bright-field images of hepatocytes (darker cells) and 3T3 fibroblasts cultured on the comb fingers. (D) Devices in a standard 12-well plate. Cell culture and functional assays were performed with standard methods. Figure adapted from Hui et al. (2007).
This study indicated that ESC colony size can impact cell fate decisions, suggesting the importance of controlling this parameter. One technology to modulate ESC colony is the Bio Flip Chip (BFC), a polymeric chip fabricated with microwells that allow for cell patterning on culture substrates or other cell layers (Rosenthal et al., 2007). Cells are pipetted on the surface of the BFC and allowed to settle into microwells. The chip is then inverted onto the desired culture substrate, allowing the cells to settle, attach, and grow in the desired pattern. By altering the spacing between microwells, the chip can be used to investigate the effects of cell density and cell-cell contact on stem cell culture. Using this technology, Rosenthal et al. found that seeding mouse ESCs as single cells maximized the number of resulting undifferentiated colonies. This behavior is distinct from that of human ESCs, which have low survival rates as single cells (Stewart et al., 2006). Therefore, these studies demonstrate that modulating cell density can impact and potentially control cell fate, but in a cell-specific fashion.
Cell density is an important variable not only for the self-renewal but also for the differentiation of embryonic stem cells. The first steps of lineage commitment of embryonic stem cells can occur in embryoid bodies (EBs), which express markers of all three embryonic germ layers. EB formation is typically conducted in suspension culture via growth factor removal, which results in spheroids with a broad size distribution. The heterogeneity in EB size can impact further downstream differentiation through cell density and diffusion effects, among others. To allow for more precise control over embryoid body formation, Karp et al. created microfabricated polymeric microwells for ESC differentiation that allow for control over EB size through variation of microwell dimensions (Karp et al., 2007). Murine EBs generated using this method had more uniform expression of α-fetoprotein, brachyury, and nestin – markers of the three embryonic germ layers – a result that may impact the efficiency of downstream differentiation processes.
Direct cell-cell contact
Stem cells have important interactions with the heterogeneous cell types that comprise stem cell niches, among them direct cell-cell signaling relationships through molecules expressed on the cell surface. Presentation of the signaling molecules in a fluid lipid bilayer differs from ECM-immobilization, for instance, in that it allows for diffusion and receptor clustering. Clustering of cell surface receptors often serves to polarize cells and in some cases is necessary for activation of downstream signaling pathways. Integrins, the major class of cell surface adhesion proteins, are well known to mediate signaling through receptor clustering. Phosphorylation of downstream signaling targets depends on β1 integrin clustering (Kornberg et al., 1992), and presentation of synthetic integrin ligands in clusters reduced the ligand density required for cell adhesion (Maheshwari et al., 2000). Similarly, antibody-induced oligomerization of soluble, recombinant Notch ligand Delta is in many cases necessary for receptor binding, internalization, and activation of downstream signaling targets and has been used to activate Notch in neural crest stem cells (Hicks et al., 2002; Morrison et al., 2000).
Presentation of membrane-bound signals is also a mechanism by which cell polarity is established in stem cell niches. Study of the development of the germline in Drosophila has led to major insights into the role of the niche in directing cell fate decisions, and in particular the signals provided by neighboring cells. In the male germline stem cell (GSC) niche, one role of hub cells is to establish stem cell polarity (Yamashita et al., 2005). Stem cell polarity is necessary for asymmetric cell division, which is the primary mechanism of self-renewal. Adherens junctions between GSCs and hub cells enable orthogonal orientation of the stem cell mitotic spindle with respect to the hub, an elegant illustration of how niche cells guide stem cell fate decisions (Yamashita et al., 2003).
These examples of how membrane-bound or lipophilic factors can regulate cell function are particularly instructive for the design of stem cell microenvironments. As shown above, soluble ligands often do not result in the desired downstream effects. Thus, one successful approach in designing synthetic microenvironments for stem cell culture has been to incorporate ordinarily lipid-bound signaling molecules into the surface of biomaterials, thereby mimicking the native cell-cell interactions.
The morphogen Shh has important roles in development, and is thus a critical factor in stem cell engineering efforts. Lipid modifications at both the N- and C-termini of Shh enable interactions with the transmembrane protein Dispatched that lead to Shh multimerization (Kawakami et al., 2002; Zeng et al., 2001). Motivated by the biochemistry of naturally occurring Shh, immobilization and/or oligomerization of Shh molecules on biomaterials in a manner that allowed for multivalency was explored as a technique to stimulate angiogenesis in a chick corioallantoic membrane assay (Wall et al., 2008). Multivalent, immobilized Shh had a more potent angiogenic effect than soluble Shh, illustrating how multimerization of lipophilic signaling molecules can be effectively emulated ex vivo.
The Notch signaling pathway is also broadly implicated in the regulation of numerous stem cell populations (Carlson et al., 2007); therefore, there is considerable interest in modulating the activity of this pathway in culture to regulate cell fate. Liu and colleagues have designed artificial ECM proteins that express the bioactive domain of Notch ligands Jagged and Delta for use in neural stem cell culture (Liu et al., 2003; Liu et al., 2003). Similarly, microbeads functionalized with immobilized Notch ligands were used to direct differentiation of bone marrow-derived hematopoietic stem cells into T cells (Taqvi et al., 2006). Addition of the functionalized beads to an established lymphoid differentiation co-culture system shifted the balance of differentiated cells from exclusively B cells in the absence of Notch signaling to a mix of B and T cells, indicating that recreating the signaling environment normally provided by neighboring cells can recapitulate the niche ex vivo. This system can also be used to study effects of ligand density on downstream cell fates, providing additional design criteria for stem cell engineers.
Synthetic membranes have also been explored as a method to recreate cell-cell interactions ex vivo. In these systems, cells are cultured on a supported lipid bilayer presenting a signaling molecule. Using this technique to explore cell-cell interactions at the neuronal synapse, Groves, Isacoff, and colleagues report that clustering of signaling molecules in the membrane lead to strengthening of cell-membrane adhesion (Pautot et al., 2005). This work reconstituting the neuronal synapse with a synthetic lipid bilayer can be extended to stem cell applications.
ECM presents important biochemical and mechanical cues to stem cells
The specific cell-cell interactions and growth factors that influence stem cell fates in vivo exist in combination with signals from the extracellular matrix. ECM has been primarily understood as the adhesive substrate that anchors cells within their microenvironments. For example, in the Drosophila GSC niche, abrogation of hub cell adhesion to the ECM resulted in mislocalization of hub cells and subsequent disruption of the mechanisms by which hub cells support GSC self-renewal (Tanentzapf et al., 2007). However, the ECM itself can also provide instructive cues for cell fate decisions, primarily via the integrin family of cell surface adhesion receptors. In erythropoiesis, for example, adhesion of primary erythroid progenitors to the niche ECM protein fibronectin mediated by α4β1 integrin is necessary for proper proliferation in vitro (Eshghi et al., 2007). In this system, signals from the ECM thus cooperate with signals from the soluble factor erythropoietin to activate signaling pathways necessary for terminal differentiation and proliferation.
ECM molecules are typically quite large and present diverse domains for cell adhesion. In addition, many ECM proteins such as fibronectin and laminin are characterized by alternative splicing events as well as post-translational modifications, making it sometimes difficult to work with purified proteins. Synthetic ECMs, comprised of polymer backbones functionalized with adhesion motifs, have thus been utilized to dissect the mechanisms by which ECMs support stem cell fate decisions. For example, the ECM molecule laminin, which is important in both in vivo and in vitro neural stem cell (NSC) niches, engages at least 7 distinct integrin receptor pairs (Powell and Kleinman, 1997). In order to achieve greater control over NSC fate, synthetic ECMs incorporating several laminin motifs were designed and used to elucidate the differential effects of adhesion molecule motifs on stem cell fate decisions (Saha et al., 2007). This work demonstrated that, surprisingly, the canonical integrin adhesion sequence RGD was sufficient to support the proliferation and differentiation of NSCs, whereas other laminin-derived adhesion motifs were unable to support these stem cell functions.
The ECM in a given tissue typically exists as a mix of several different proteins – often collagens, laminins, and fibronectins – and high-throughput approaches have accordingly been developed to investigate the combinatorial effects of ECMs on stem cell fates. One system analyzed the combinatorial effects of ECM on differentiation of ESCs into hepatocytes, and it found that an approximately 140-fold difference between the least and most efficient conditions, suggesting that ECM components may strongly influence hepatic differentiation (Flaim et al., 2005). This work has been extended to include synthetic ECMs in addition to various soluble growth factors, yielding the interesting result that the effect of a given growth factor often depended on the substrate background in which it is presented (Nakajima et al., 2007).
In addition to biochemical motifs or signals, the ECM also provides a physical framework in which stem cells reside and develop. Importantly, the diverse tissues of the adult body exhibit a range of matrix stiffness spanning several orders of magnitude, and such differences in substrate stiffness have long been known to influence cell fate decisions in differentiated cell types (Discher et al., 2005). An emerging area of study in stem cell biology and engineering is investigation of the role of these mechanical cues in stem cell fate decisions.
Because mesenchymal stem cells can differentiate in vitro into cell types from tissues ranging from muscle, bone, and potentially brain, Engler and colleagues hypothesized that the mechanical cues provided by the ECM are particularly instructive in lineage specification. Their study provides evidence that matrix elasticity influences differentiation of human MSCs into osteogenic, myogenic, and neurogenic cells (Engler et al., 2006). Specifically, by culturing naïve MSCs on elastically-tunable polyacrylamide gels, they show that gels of bone-like stiffness led to osteogenic differentiation, gels of muscle-like stiffness resulted in myogenic differentiation and soft gels of brain-like stiffness led to neuronal differentiation.
This landmark work illustrates the principle that stem cells are regulated by the mechanics of the cellular microenvironment. Mesenchymal stem cells generate lineages that are present in load-bearing tissue, and it would therefore be interesting to determine whether this important principle extends to other stem cell types, including ones from tissues that are relatively mechanically insulated. It has recently been found that substrate stiffness collaborates with soluble medium conditions to regulate the proliferation and differentiation of adult neural stem cells (Saha et al., submitted). Cells exhibit an optimum in proliferation (in FGF-2) and an optimum in neuronal differentiation (in retinoic acid) at an intermediate stiffness that is characteristic of brain tissue. Furthermore, under conditions that induce general or nonspecific cell differentiation (serum), stiff substrates support the differentiation of GFAP-expressing astrocytes, whereas soft substrates preferentially support the differentiation of β-III-tubulin expressing neurons. Taken together, these results suggest that the design of ex vivo stem cell culture systems should consider mechanical cues in the microenvironment such as matrix stiffness as factors in guiding proper lineage specification.
Summary
Stem cell behavior is controlled by the interactions of the soluble and immobilized factors, heterogeneous cell types, and extracellular matrix molecules that collectively comprise the stem cell niche. Furthermore, these components vary in a spatiotemporal fashion. Progress toward culturing and expanding stem cells outside their native environments depends on identification and mechanistic understanding of the contributions of these components, as well as technologies to control the presentation of multiple signals to cells. This chapter has reviewed diverse mechanisms by which niche components influence stem cell fate decisions, as well as presented successful efforts to incorporate these concepts into the design of biomimetic stem cell microenvironments.
Future progress in the field of synthetic stem cell microenvironments will be aided by a detailed, mechanistic understanding of the biology of native stem cell niches. In particular, understanding the role of niche components in instructing specific cell fate decisions versus selecting among heterogeneous cells is of critical importance. In addition, cell fate decisions are influenced by a number of factors in combination. To this end, systems biology approaches that can detect combinatorial interactions are of strong interest. Finally, novel technologies that enable the delivery of extrinsic stem cell fate determinants along with approaches to vary intrinsic factors will allow for the development of truly biomimetic environments that yield exquisite control over stem cell fates.
Acknowledgements
This work was funded by UC Discovery bio05-10559 and the UC Chancellor’s Faculty Partnership Fund.
References
- Austin C.P, Feldman D.E, Ida J.A Jr, Cepko C.L. Vertebrate retinal ganglion cells are selected from competent progenitors by the action of Notch. Development. 1995;121:3637–3650. [PubMed: 8582277]
- Bartlett P.F, Brooker G.J, Faux C.H, Dutton R, Murphy M, Turnley A, Kilpatrick T.J. Regulation of neural stem cell differentiation in the forebrain. Immunol Cell Biol. 1998;76:414–418. [PubMed: 9797460]
- Bendall S.C, Stewart M.H, Menendez P, George D, Vijayaragavan K, Werbowetski-Ogilvie T, Ramos-Mejia V, Rouleau A, Yang J, Bosse M. et al. IGF and FGF cooperatively establish the regulatory stem cell niche of pluripotent human cells in vitro. Nature. 2007;448:1015–1021. [PubMed: 17625568]
- Bhushan A, Itoh N, Kato S, Thiery J.P, Czernichow P, Bellusci S, Scharfmann R. Fgf10 is essential for maintaining the proliferative capacity of epithelial progenitor cells during early pancreatic organogenesis. Development. 2001;128:5109–5117. [PubMed: 11748146]
- Briscoe J, Ericson J. Specification of neuronal fates in the ventral neural tube. Curr Opin Neurobiol. 2001;11:43–49. [PubMed: 11179871]
- Carlson M.E, O’Connor M.S, Hsu M, Conboy I.M. Notch signaling pathway and tissue engineering. Front Biosci. 2007;12:5143–5156. [PubMed: 17569636]
- D’Amour K.A, Agulnick A.D, Eliazer S, Kelly O.G, Kroon E, Baetge E.E. Efficient differentiation of human embryonic stem cells to definitive endoderm. Nat Biotechnol. 2005;23:1534–1541. [PubMed: 16258519]
- D’Amour K.A, Bang A.G, Eliazer S, Kelly O.G, Agulnick A.D, Smart N.G, Moorman M.A, Kroon E, Carpenter M.K, Baetge E.E. Production of pancreatic hormone-expressing endocrine cells from human embryonic stem cells. Nat Biotechnol. 2006;24:1392–1401. [PubMed: 17053790]
- Discher D.E, Janmey P, Wang Y.L. Tissue cells feel and respond to the stiffness of their substrate. Science. 2005;310:1139–1143. [PubMed: 16293750]
- Engler A.J, Sen S, Sweeney H.L, Discher D.E. Matrix elasticity directs stem cell lineage specification. Cell. 2006;126:677–689. [PubMed: 16923388]
- Eshghi S, Vogelezang M.G, Hynes R.O, Griffith L.G, Lodish H.F. Alpha4beta1 integrin and erythropoietin mediate temporally distinct steps in erythropoiesis: integrins in red cell development. J Cell Biol. 2007;177:871–880. [PMC free article: PMC2064286] [PubMed: 17548514]
- Fan V.H, Tamama K, Au A, Littrell R, Richardson L.B, Wright J.W, Wells A, Griffith L.G. Tethered epidermal growth factor provides a survival advantage to mesenchymal stem cells. Stem Cells. 2007;25:1241–1251. [PubMed: 17234993]
- Flaim C.J, Chien S, Bhatia S.N. An extracellular matrix microarray for probing cellular differentiation. Nat Methods. 2005;2:119–125. [PubMed: 15782209]
- Fortunel N.O, Otu H.H, Ng H.H, Chen J, Mu X, Chevassut T, Li X, Joseph M, Bailey C, Hatzfeld J.A. et al. Comment on “‘Stemness’: transcriptional profiling of embryonic and adult stem cells” and “a stem cell molecular signature” Science. 2003;302:393. author reply 393. [PubMed: 14563990]
- Gu G, Brown J.R, Melton D.A. Direct lineage tracing reveals the ontogeny of pancreatic cell fates during mouse embryogenesis. Mech Dev. 2003;120:35–43. [PubMed: 12490294]
- Hebrok M, Kim S.K, Melton D.A. Notochord repression of endodermal Sonic hedgehog permits pancreas development. Genes Dev. 1998;12:1705–1713. [PMC free article: PMC316875] [PubMed: 9620856]
- Hicks C, Ladi E, Lindsell C, Hsieh J.J, Hayward S.D, Collazo A, Weinmaster G. A secreted Delta1-Fc fusion protein functions both as an activator and inhibitor of Notch1 signaling. J Neurosci Res. 2002;68:655–667. [PubMed: 12111827]
- Ho J.E, Chung E.H, Wall S, Schaffer D.V, Healy K.E. Immobilized sonic hedgehog N-terminal signaling domain enhances differentiation of bone marrow-derived mesenchymal stem cells. J Biomed Mater Res A. 2007;83:1200–1208. [PubMed: 17600327]
- Hui E.E, Bhatia S.N. Micromechanical control of cell-cell interactions. Proc Natl Acad Sci U S A. 2007;104:5722–5726. [PMC free article: PMC1851558] [PubMed: 17389399]
- Ivanova N.B, Dimos J.T, Schaniel C, Hackney J.A, Moore K.A, Lemischka I.R. A stem cell molecular signature. Science. 2002;298:601–604. [PubMed: 12228721]
- Karp J.M, Yeh J, Eng G, Fukuda J, Blumling J, Suh K.Y, Cheng J, Mahdavi A, Borenstein J, Langer R, Khademhosseini A. Controlling size, shape and homogeneity of embryoid bodies using poly(ethylene glycol) microwells. Lab Chip. 2007;7:786–794. [PubMed: 17538722]
- Kawakami T, Kawcak T, Li Y.J, Zhang W, Hu Y, Chuang P.T. Mouse dispatched mutants fail to distribute hedgehog proteins and are defective in hedgehog signaling. Development. 2002;129:5753–5765. [PubMed: 12421714]
- Kim S.K, Hebrok M, Melton D.A. Notochord to endoderm signaling is required for pancreas development. Development. 1997;124:4243–4252. [PubMed: 9334273]
- Kornberg L, Earp H.S, Parsons J.T, Schaller M, Juliano R.L. Cell adhesion or integrin clustering increases phosphorylation of a focal adhesion-associated tyrosine kinase. J Biol Chem. 1992;267:23439–23442. [PubMed: 1429685]
- Kuhl P.R, Griffith-Cima L.G. Tethered epidermal growth factor as a paradigm for growth factor-induced stimulation from the solid phase. Nat Med. 1996;2:1022–1027. [PubMed: 8782461]
- Lammert E, Cleaver O, Melton D. Induction of pancreatic differentiation by signals from blood vessels. Science. 2001;294:564–567. [PubMed: 11577200]
- Li Y, Zhang H, Litingtung Y, Chiang C. Cholesterol modification restricts the spread of Shh gradient in the limb bud. Proc Natl Acad Sci U S A. 2006;103:6548–6553. [PMC free article: PMC1458921] [PubMed: 16611729]
- Liu C.Y, Apuzzo M.L, Tirrell D.A. Engineering of the extracellular matrix: working toward neural stem cell programming and neurorestoration–concept and progress report. Neurosurgery. 2003;52:1154–1165. discussion 1165–1157. [PubMed: 12699561]
- Liu C.Y, Westerlund U, Svensson M, Moe M.C, Varghese M, Berg-Johnsen J, Apuzzo M.L, Tirrell D.A, Langmoen I.A. Artificial niches for human adult neural stem cells: possibility for autologous transplantation therapy. J Hematother Stem Cell Res. 2003;12:689–699. [PubMed: 14977478]
- Lowry W.E, Richter L. Signaling in adult stem cells. Front Biosci. 2007;12:3911–3927. [PubMed: 17485347]
- Maheshwari G, Brown G, Lauffenburger D.A, Wells A, Griffith L.G. Cell adhesion and motility depend on nanoscale RGD clustering. J Cell Sci. 2000;113:(Pt 10), 1677–1686. [PubMed: 10769199]
- Martin G.R. Isolation of a pluripotent cell line from early mouse embryos cultured in medium conditioned by teratocarcinoma stem cells. Proc Natl Acad Sci U S A. 1981;78:7634–7638. [PMC free article: PMC349323] [PubMed: 6950406]
- Molofsky A.V, Pardal R, Morrison S.J. Diverse mechanisms regulate stem cell self-renewal. Curr Opin Cell Biol. 2004;16:700–707. [PubMed: 15530784]
- Morrison S.J, Perez S.E, Qiao Z, Verdi J.M, Hicks C, Weinmaster G, Anderson D.J. Transient Notch activation initiates an irreversible switch from neurogenesis to gliogenesis by neural crest stem cells. Cell. 2000;101:499–510. [PubMed: 10850492]
- Munoz-Sanjuan I, Brivanlou A.H. Neural induction, the default model and embryonic stem cells. Nat Rev Neurosci. 2002;3:271–280. [PubMed: 11967557]
- Nakajima M, Ishimuro T, Kato K, Ko I.K, Hirata I, Arima Y, Iwata H. Combinatorial protein display for the cell-based screening of biomaterials that direct neural stem cell differentiation. Biomaterials. 2007;28:1048–1060. [PubMed: 17081602]
- Pautot S, Lee H, Isacoff E.Y, Groves J.T. Neuronal synapse interaction reconstituted between live cells and supported lipid bilayers. Nat Chem Biol. 2005;1:283–289. [PMC free article: PMC1448216] [PubMed: 16408058]
- Peerani R, Rao B.M, Bauwens C, Yin T, Wood G.A, Nagy A, Kumacheva E, Zandstra P.W. Niche-mediated control of human embryonic stem cell self-renewal and differentiation. Embo J. 2007;26:4744–4755. [PMC free article: PMC2080799] [PubMed: 17948051]
- Powell S.K, Kleinman H.K. Neuronal laminins and their cellular receptors. Int J Biochem Cell Biol. 1997;29:401–414. [PubMed: 9202420]
- Ramalho-Santos M, Yoon S, Matsuzaki Y, Mulligan R.C, Melton D.A. “Stemness”: transcriptional profiling of embryonic and adult stem cells. Science. 2002;298:597–600. [PubMed: 12228720]
- Reddy C.C, Niyogi S.K, Wells A, Wiley H.S, Lauffenburger D.A. Engineering epidermal growth factor for enhanced mitogenic potency. Nat Biotechnol. 1996;14:1696–1699. [PubMed: 9634854]
- Rider C.C. Heparin/heparan sulphate binding in the TGF-beta cytokine superfamily. Biochem Soc Trans. 2006;34:458–460. [PubMed: 16709187]
- Rosenthal A, Macdonald A, Voldman J. Cell patterning chip for controlling the stem cell microenvironment. Biomaterials. 2007;28:3208–3216. [PMC free article: PMC1929166] [PubMed: 17434582]
- Saha K, Irwin E.F, Kozhukh J, Schaffer D.V, Healy K.E. Biomimetic interfacial interpenetrating polymer networks control neural stem cell behavior. J Biomed Mater Res A. 2007;81:240–249. [PubMed: 17236216]
- Saha K, Schaffer D.V. Signal dynamics in Sonic hedgehog tissue patterning. Development. 2006;133:889–900. [PubMed: 16452094]
- Sakiyama-Elbert S.E, Hubbell J.A. Development of fibrin derivatives for controlled release of heparin-binding growth factors. J Control Release. 2000;65:389–402. [PubMed: 10699297]
- Schofield R. The relationship between the spleen colony-forming cell and the haemopoietic stem cell. Blood Cells. 1978;4:7–25. [PubMed: 747780]
- Stewart M.H, Bosse M, Chadwick K, Menendez P, Bendall S.C, Bhatia M. Clonal isolation of hESCs reveals heterogeneity within the pluripotent stem cell compartment. Nat Methods. 2006;3:807–815. [PubMed: 16990813]
- Takahashi K, Tanabe K, Ohnuki M, Narita M, Ichisaka T, Tomoda K, Yamanaka S. Induction of pluripotent stem cells from adult human fibroblasts by defined factors. Cell. 2007;131:861–872. [PubMed: 18035408]
- Takahashi K, Yamanaka S. Induction of pluripotent stem cells from mouse embryonic and adult fibroblast cultures by defined factors. Cell. 2006;126:663–676. [PubMed: 16904174]
- Tanentzapf G, Devenport D, Godt D, Brown N.H. Integrin-dependent anchoring of a stem-cell niche. Nat Cell Biol. 2007;9:1413–1418. [PMC free article: PMC3529653] [PubMed: 17982446]
- Taqvi S, Dixit L, Roy K. Biomaterial-based notch signaling for the differentiation of hematopoietic stem cells into T cells. J Biomed Mater Res A. 2006;79:689–697. [PubMed: 16845670]
- Thomson J.A, Itskovitz-Eldor J, Shapiro S.S, Waknitz M.A, Swiergiel J.J, Marshall V.S, Jones J.M. Embryonic stem cell lines derived from human blastocysts. Science. 1998;282:1145–1147. [PubMed: 9804556]
- Wall S.T, Saha K, Ashton R.S, Kam K.R, Schaffer D.V, Healy K.E. Multivalency of Sonic Hedgehog Conjugated to Linear Polymer Chains Modulates Protein Potency. Bioconjug Chem. 2008 [PubMed: 18380472]
- Wichterle H, Lieberam I, Porter J.A, Jessell T.M. Directed differentiation of embryonic stem cells into motor neurons. Cell. 2002;110:385–397. [PubMed: 12176325]
- Willerth S.M, Rader A.R, Sakiyama-Elbert S.E. The Effect of Controlled Growth Factor Delivery on Embryonic Stem Cell Differentiation Inside of Fibrin Scaffolds. Stem Cell Research. 2008 in press . [PMC free article: PMC2744946] [PubMed: 19383401]
- Wright D, Rajalingam B, Selvarasah S, Dokmeci M.R, Khademhosseini A. Generation of static and dynamic patterned co-cultures using microfabricated parylene-C stencils. Lab Chip. 2007;7:1272–1279. [PubMed: 17896010]
- Wu H.H, Ivkovic S, Murray R.C, Jaramillo S, Lyons K.M, Johnson J.E, Calof A.L. Autoregulation of neurogenesis by GDF11. Neuron. 2003;37:197–207. [PubMed: 12546816]
- Yamashita Y.M, Fuller M.T, Jones D.L. Signaling in stem cell niches: lessons from the Drosophila germline. J Cell Sci. 2005;118:665–672. [PubMed: 15701923]
- Yamashita Y.M, Jones D.L, Fuller M.T. Orientation of asymmetric stem cell division by the APC tumor suppressor and centrosome. Science. 2003;301:1547–1550. [PubMed: 12970569]
- Zeng X, Goetz J.A, Suber L.M, Scott W.J Jr, Schreiner C.M, Robbins D.J. A freely diffusible form of Sonic hedgehog mediates long-range signalling. Nature. 2001;411:716–720. [PubMed: 11395778]
- *
Edited by: Sangeeta Bhatia and Julia Polak. Last revised June 3, 2008. Published September 15, 2008. This chapter should be cited as: Eshghi, S. and Schaffer, D.V., Engineering microenvironments to control stem cell fate and function (September 15, 2008), StemBook, ed. The Stem Cell Research Community, StemBook, doi/10.3824/stembook.1.5.1, http://www.stembook.org.
- Challenges and Opportunities to Harnessing the (Hematopoietic) Stem Cell Niche.[Curr Stem Cell Rep. 2016]Challenges and Opportunities to Harnessing the (Hematopoietic) Stem Cell Niche.Choi JS, Harley BA. Curr Stem Cell Rep. 2016 Mar; 2(1):85-94. Epub 2016 Jan 29.
- Bioengineering of the Human Neural Stem Cell Niche: A Regulatory Environment for Cell Fate and Potential Target for Neurotoxicity.[Results Probl Cell Differ. 2018]Bioengineering of the Human Neural Stem Cell Niche: A Regulatory Environment for Cell Fate and Potential Target for Neurotoxicity.Buzanska L, Zychowicz M, Kinsner-Ovaskainen A. Results Probl Cell Differ. 2018; 66:207-230.
- Biomimetic three-dimensional microenvironment for controlling stem cell fate.[Interface Focus. 2011]Biomimetic three-dimensional microenvironment for controlling stem cell fate.Zhang H, Dai S, Bi J, Liu KK. Interface Focus. 2011 Oct 6; 1(5):792-803. Epub 2011 Jul 27.
- Review Designing stem cell niches for differentiation and self-renewal.[J R Soc Interface. 2018]Review Designing stem cell niches for differentiation and self-renewal.Donnelly H, Salmeron-Sanchez M, Dalby MJ. J R Soc Interface. 2018 Aug; 15(145).
- Review Presentation counts: microenvironmental regulation of stem cells by biophysical and material cues.[Annu Rev Cell Dev Biol. 2010]Review Presentation counts: microenvironmental regulation of stem cells by biophysical and material cues.Keung AJ, Kumar S, Schaffer DV. Annu Rev Cell Dev Biol. 2010; 26:533-56.
- Engineering microenvironments to control stem cell fate and function - StemBookEngineering microenvironments to control stem cell fate and function - StemBook
- Cellular and nuclear reprogramming - StemBookCellular and nuclear reprogramming - StemBook
Your browsing activity is empty.
Activity recording is turned off.
See more...