This is an open-access article distributed under the terms of the Creative Commons Attribution License, which permits unrestricted use, distribution, and reproduction in any medium, provided the original work is properly cited.
NCBI Bookshelf. A service of the National Library of Medicine, National Institutes of Health.
StemBook [Internet]. Cambridge (MA): Harvard Stem Cell Institute; 2008-. doi: 10.3824/stembook.1.33.1
Clonal marking methods represent one of the most powerful tools available for identifying stem cells and analyzing their function. Derived from classical approaches in cell lineage tracing during development, we review modern cell lineage methods and describe how they are used to identify stem cells and their niches. We discuss key considerations a stem cell researcher must be aware of to avoid some of the common pitfalls of lineage studies. Finally, using basic knowledge of the target tissue and the guidelines discussed herein, we explain how raw lineage information can be converted into sound conclusions about the number, location, behavior and regulation of tissue stem cells.
Introduction
Adult metazoan tissues are complex, dynamic structures whose maintenance depends on many of the same processes that guide developing embryos. While some cells in a typical tissue may persist indefinitely, many more live only briefly and are replaced by processes of repair and renewal that require new cells to arise and differentiate. Often, the ultimate sources of rejuvenation are stem cells, rare cells that are defined by their ability to both produce daughters and self-renew, frequently by dividing asymmetrically. Although stem cells are critically important for tissue health and homeostasis, finding these cells and characterizing their cellular properties is difficult. Tissues contain so many different cells and cell types, in complex three-dimensional relationships, and undergoing complex movements that it is generally impossible to identify stem cells histologically. Patterns of gene expression, like classical histological stains, are essential markers in the quest to understand tissue biology, but are entirely insufficient to reliably identify stem cells or to understand tissue dynamics.
Lineage analysis, a technique originally developed to study early embryos, represents by far the most powerful and reliable tool for identifying stem cells and for deciphering other aspects of tissue behavior. Ideally, a single cell is marked in such a manner that the mark is faithfully transmitted to all daughters of the initial cell, resulting in a labeled clone. By analyzing the number, location and phenotype of clonally derived cells at increasingly greater times after marking, the types of cells generated by the progenitor, their movements and their lifetimes can all be deduced. Moreover, a cell's clonal profile reveals information about its state of differentiation. In the absence of extensive cell mixing, a thorough analysis of all the clonal types produced by a tissue allows the complexity of its component cell types and their movements to be determined. However, like the generation of a 3D protein structure from its diffraction patterns, constructing an accurate picture of a tissue's dynamics from its lineage patterns is complex, non-intuitive, and fraught with potential problems. How this process is carried out forms the subject of this Chapter.
For the purposes of stem cell studies, the key step in lineage analysis is identifying stem cell clones. Most dividing cells that are marked in a lineage experiment are not stem cells. Non-stem cells generate clones known as transient clones, that tend to be variable in size because they commence at every point along the lineage. Transient clones are short-lived and mature to contain only differentiated cells (Movie 1). In contrast, when the initial marked cell is a stem cell, it will display different properties. Stem cell clones tend to be larger and more uniform in size because these clones all commence at a single upstream position in the lineage. In addition, stem cell clones persist longer than transient clones and always contain undifferentiated, intermediate and differentiated progeny (Movie 2).
Once stem cell clones have been recognized, the location of the stem cell can be determined by studying their pattern of growth with time. However, much more can be learned besides just stem cell identity. Studying stem cell lineages over time also provides the key to understanding stem cell potency, lifetime, replacement, interconversion, and aging. Analyzing mutant genes within stem cell clones represents the critical test of whether and how particular genetic pathways control stem cell behavior. Finally, clonally analyzing stem cells is an essential prerequisite for discovering and characterizing the tissue niches that play an equal role with stem cells in ensuring a stable supply of replacement cells throughout the life of a tissue.
Movie 1Transient clone
In this hypothetical tissue, four stem cells (highlighted in dark blue at the beginning of the movie) are maintained in a niche (blue gradient highlighted in light blue at the beginning of the movie) produced by a population of non-dividing niche-generating cells (purple, highlighted in red at the beginning of the movie). Each stem cell daughter undergoes three transient divisions. The lineage pattern of a transient clone induced in the non-stem cell daughter is shown. Label (red) appears first in the recombined cell, moves toward the edge as cells divide and then turns over. Expansion and eventual loss of a labeled clone when the label (red) is acquired by the earliest non-stem cell daughter of a lineage. If the initial clone induction event occurs at later points in the lineage, the clone will still be lost following sufficient cell turnover, but only the fraction of the lineage directly descended from the initial labeled cell will acquire label.
Movie 2Stem cell clone
In this hypothetical tissue, four stem cells (highlighted in dark blue at the beginning of the movie) are maintained in a niche (blue gradient highlighted in light blue at the beginning of the movie) produced by a population of non-dividing niche-generating cells (purple, highlighted in red at the beginning of the movie). Each stem cell daughter undergoes three transient divisions. The lineage pattern of a stem cell clone is shown. Label (red) appears first in the recombined stem cell, spreads toward the edge as cells divide, but remains in all cells and does not turn over.
Lineage tracing methods
Direct observation
C.O. Whitman and his followers, E.G. Wilson, A.L. Conklin and F.R. Lillie carried out the first lineage tracing experiments at the end of the 19th century (Whitman, 1898; Stent and Weisblat, 1985). To determine whether the fate of cells arising from each blastomere of annelids, ascidians and mollusks were predetermined they used direct observation of living embryos. Direct observation has been used with great success in the nematode, C. elegans where the entire course of embryonic development has been fate mapped (Sulston et al., 1983). Many cells were found to divide for a period in a stem cell-like manner (Chalfie et al., 1981). While direct observation has the advantage that it is non-invasive, it is currently applicable only to relatively small groups of cells that are present in an optically clear tissue. However, progress is currently being made in the use of time-lapse microscopy and this is likely to become an increasingly important method of analyzing stem cell lineages.
Marker addition
Cells have been directly marked for lineage tracing using many different reagents, including vital dyes, radioactive molecules and intracellular tracers. Vital dyes that stain living cells were first used to follow cell lineage in the amphibian embryo by applying dyes to the surface of cells (Vogt, 1924; Vogt, 1929), however, the dyes used in these experiments were water soluble and therefore prone to spread to neighboring cells. The discovery of lipid soluble dyes, DiI and DiO, that are incorporated into cell membranes and give a high intensity of fluorescence solved this problem (Axelrod, 1979). Lineage tracing by radioactive labeling involves soaking the cells or tissue in tritiated thymidine and then grafting them back into the host tissue. This technique was first used to study early cardiac differentiation in the chick (Rosenquist, 1966). Intracellular tracers are introduced into cells by injection, ballistic techniques or electroporation, which are less invasive than grafting. They have been used in a wide range of species including mapping the fate of cells of the mouse epiblast at gastrulation (Lawson and Pedersen, 1986). The intracellular tracers commonly used have been horseradish peroxidase and fluorophores conjugated to dextran (to prevent their movement through gap junctions). However, all direct cell labeling methods have the disadvantage that the label is diluted upon cell division and is therefore of little use for long-term lineage analysis.
Retroviral infection
Replication defective retroviruses carrying marker genes (most commonly horse radish peroxidase or β-galactosidase) infect cells and integrate into their genome, thereby allowing the infected cells and all their progeny to be followed over time. This technique was first used to trace cells of the murine retina (Price and D Cepko, 1987) and has since been used in a wide variety of species and tissues including hematopoietic stem cells (Lemischka et al., 1986; Jordan and Lemischka, 1990) and lung progenitor cells (Engelhardt et al., 1995). It has many advantages as infection is relatively innocuous, does not spread to neighboring cells and the retrovirus is passed on from mother to daughter. Additionally, since the retrovirus contains a drug resistance cassette, infected cells can be selected for growth in culture. However, clonal boundaries are often hard to define as infection of adjacent cells can occur. Efforts to address this issue have utilized retrovirus libraries, in which each retrovirus has a unique DNA tag thereby dramatically reducing the possibility of adjacent cells being infected with the same retrovirus (Golden et al., 1995). However, in order to determine whether a labeled patch is a single clone, the cells have to be excised and assayed by PCR.
Genetic alteration
Using genetic differences between cells to trace lineages has a long history and these methods all have the huge advantage that the marker is inherited by all daughter cells.
Genetic mosaics
Genetic mosaics have been used to carry out lineage analysis in a wide range of species. Beginning in 1929, flies mosaic for male and female cells (gyandromorphs) have been used to fate map Drosophila embryonic cells (Sturtevant, 1929; Garcia-Bellido and Merriam, 1969). In mice, females hemizygous for a β-galactosidase transgene on the X chromosome have been used to study the behavior of limbal stem cell clones (Collinson et al., 2002). This technique has the disadvantage that many cells are initially marked and the results can therefore be hard to interpret. Natural genetic mosaics can arise in human tumors, leading to the proposal in 1875 that cancer arises clonally (Cohnheim, 1875). The clonality of a wide range of human cancers has since been studied by following cells marked by chromosomal translocations, retroviral integration sites and the expression of X-linked markers (Garcia et al., 2000). Unfortunately, lineage analysis has not yet been widely used to address the existence of cancer stem cells.
Transplantation
Transplantation of cells can also create genetic mosaics. This has been carried out extensively in two systems: during chick development and in the study of the hematopoietic stem cells in adult mice. The discovery that the first hematopoietic stem cells arise in the yolk sac involved creating chick genetic mosaics by establishing parabiosis between male and female embryos (Moore and Owen, 1965). Alternatively, chick and quail cells can be distinguished by heterochromatin morphology. Grafting of quail tissue into chick embryos can therefore be used for lineage tracing (Le Douarin and Barq, 1969). Many careful experiments have been carried out using this method including following the migration of the neural crest cells and deducing their contribution to various tissues.
Mosaics generated by cell transplantation have been widely used to study stem cells in mice. Following introduction into the circulation, hematopoietic stem cells are able to populate the bone marrow and the hematopoietic system for long periods of time. The hematopoietic stem cell lineage tracing method of choice has therefore been transplantation. This technique was used to establish that the hematopoietic stem cell is capable of giving rise to both myeloid and lymphoid lineages using cells marked by radiation induced chromosomal changes (Abramson, 1979). A general method based on producing chimeras from ES cells bearing different fluorescent marker genes revealed a polyclonal origin for the yolk sac blood islands (Ueno and Weissman, 2006). While much information has been gained from experimentally generated mosaics, this method has the major disadvantage of relocating cells from their original environment to a new one, with uncertain affects on the transplanted cells.
Recombination
Generating marked and/or mutant cells by recombination has been used for many years in developmental studies (Stern, 1968; Garcia-Bellido and Merriam, 1971). Unlike techniques that rely on label injection or tissue transplantation, clones are produced in the natural tissue environment. However, high levels of radiation were initially required to obtain usable frequencies of recombination that damaged or killed many nearby cells. Nonetheless, this approach was used to determine the existence and number of germline stem cells in the Drosophila ovary (Wieschaus and Szabad, 1979).
A major advance was to generate clones harmlessly under experimental control using site-specific recombination systems from yeast or bacteria (Golic, 1991; Lakso et al., 1992). The high efficiency of clone generation not only facilitates such experiments, but also makes it possible to obtain critically important information on the cellular requirements for recombination and the initial inheritance of the recombined chromosomes (Golic and Golic, 1996; Duffy et al., 1998). While the marked cell cannot usually be determined in advance, this problem is circumvented by analyzing many clones. Current implementations of cell marking by site-specific recombination that are now being used extensively in Drosophila and mice to analyze stem cells constitute the focus of the remainder of this Chapter.
Identifying stem cells
Selecting the marking system
Many potentially useful clonal marking techniques are currently available (Tables 1 and 2). Their suitability will depend on the promoters used as well as on their chromosomal sites of insertion. When looking for new stem cells, it is advisable to first select a system that functions primarily as a lineage marker with a wide, non-biased coverage of cells. Unless the promoters are active within the targeted stem cells, whose transcriptional properties cannot be known in advance, the search will fail. Thus, heat shock or housekeeping gene promoters are to be preferred over developmentally specific promoters, which might not be active in progenitors. Later, when stem cells and niche cells have been identified and better characterized, more specific promoters will likely be useful to determine their genetic requirements.
Table 1
Strategies for non-biased lineage study
Table 2
Strategies for clonally marking a specific cell population
Strategies for non-biased lineage study
For an initial survey, a very useful system in Drosophila generates positively marked clones in any mitotically cycling cell following expression of the yeast FLP gene (“flipase”) under heat shock control (Harrison and Perrimon, 1993). In a susceptible cell, flipase-induced recombination at the FRT sites of the construct joins a ubiquitous tubulin promoter on one chromosome to a lacZ gene on the homolog (see Figure 1A). Advantages of the system include temporal control of when clones arise (by choosing the time of heat shock), low background without heat shock, selectivity for mitotically cycling cells, and efficient function in nearly all tissues and times of development. Implementation of this system in which the constructs are located near the tip of chromosome 2L (60C) has proved itself as it was used to first identify previously unknown stem cells in the Drosophila ovary (Margolis and Spradling, 1995; Decotto and Spradling, 2005) and posterior midgut (Ohlstein and Spradling, 2006). The background of spontaneous recombination appears to be higher in other implementations.
A similar approach, the “FLP-out” system, uses heat shock-FLP to convert single un-marked cells to marked cells using intra- instead of inter- chromosomal recombination (Struhl and Basler, 1993). Additional non-biased approaches that utilize heat shock-FLP in flies are discussed below and are listed in Table 1. Tissue-directed rather than ubiquitous lineage marking approaches have commonly been used in mice. However, a recent study accomplished unbiased clonal sampling by injecting recombinase at various sites to identify diverse stem cell types in the brain (Merkle et al., 2007).
Strategies targeting specific cell populations
In some cases, it is useful to target cell marking to a specific sub-population of cells, for example, to simplify the number and types of clones produced (Table 2). However, it must be kept in mind that promoters on constructs may not be active in progenitors, and often exhibit some expression in tissues outside of those predicted, due to regulatory elements or read-through transcripts at the site of insertion. In Drosophila, the yeast Gal4/UAS system (Brand and Perrimon, 1993) can be used to refine clone induction. By using specific GAL4 drivers, one can select where the FLP gene is expressed (Duffy et al., 1998). Another method allows for both spatial and temporal control over clone induction by using a hormone-dependent GAL4 system (Han et al., 2000; Nicholson et al., 2008).
In mice, hormone-dependent recombinase expression is the principal method of clonal analysis to date. In a widely used scheme, a cell-type-specific promoter expresses Cre recombinase (see Figure 1B), rather than FLP. To achieve temporal control of recombinase activity, Cre is fused to an estrogen receptor (ER; Webster et al., 1988) or progesterone receptor (PR; Nicholson et al., 2008). Such fusion proteins require the estrogen receptor ligand tamoxifen (Forde et al., 2002) or the progesterone receptor-binding drug mifepristone (Osterwalder et al., 2001; Roman et al., 2001) for recombinase activity. In this scheme, CreER-containing animals are crossed to a reporter strain such as Rosa 26R, which ubiquitously expresses a lacZ gene interrupted by an intervening Lox site-flanked stop sequence. Administering tamoxifen to these mice floxes out the intervening sequence in a fraction of the cells expressing Cre-ER, resulting in reporter expression in these cells and their descendents (see Figure 1B). If low amounts of tamoxifen are used, individual clones can be identified. This approach has proved its value in recent studies of stem cell populations within the testis (Nakagawa et al., 2007), skin (Clayton et al., 2007) and intestine (Barker et al., 2007). While Cre is the preferred recombinase in the mouse, an analogous strategy using FLP-ER is also available (Hunter et al., 2005). Conversely, Cre-lox can be used in flies, although few drivers exist and Cre toxicity may be a problem (Heidmann and Lehner, 2001; Siegal and Hartl, 1996).
Dual labeling systems
Some systems differentially label the two daughters of the cell in which recombination occurs. Such “dual label” marking is extremely helpful in deducing complex cell behavior, but analyzing the marked tissue often takes longer. In one dual labeling system (Nystul and Spradling, 2007) all cells initially express both GFP and lacZ, due to marker genes located on homologs bearing FRT sites. Upon recombination, the two markers segregate, giving rise to twin GFP positive, lacZ negative and GFP negative, lacZ positive clones. Unlike the tub-lacZ system, this system does exhibit a low background level of recombination in the absence of heat shock. A dual labeling method available in the mouse uses the mosaic analysis with double markers system (MADM; Zong et al., 2005). This system reconstitutes GFP and RFP coding sequences, which prior to recombination reside as N or C terminal halves on opposite homologs. MADM-based lineage tracing has been used to characterize the restricted fate of granular cells in the developing mouse brain (Zong et al., 2005).
Clonal analysis with mutants/mis-expression
Once a stem cell system has been identified, clone generation can be combined with genetic manipulation to facilitate functional analysis. In Drosophila, the most common method (for example, see (Dang and Perrimon, 1992; Xu and Rubin, 1993)) uses interchromosomal recombination to generate clones of mutant cells that no longer express a marker present in the rest of the cells (see Figure 1C). It is also possible to generate positively marked mutant clones, which are easier to detect in a dense tissue. The mosaic analysis with repressible cell maker method, or MARCM, facilitates the production of positively marked clones in Drosophila (Lee and Luo, 2001). This method uses Gal4/UAS and the Gal4 repressor Gal80 (Ma and Ptashne, 1987). Upon heat shock-induced mitotic recombination, Gal80-expressing cells segregate from cells homozygous for a mutation (see Figure 1D). Mutant cells contain a ubiquitous Gal4 and a UAS GFP reporter and become GFP positive following Gal80 turnover. However, MARCM clones are often slow to activate reporter expression due to the slow decay of Gal80. MARCM can also be used for clonal transgene overexpression. Transgene overexpression in flies can also be accomplished by the positively marked mosaic lineage system (Kirilly et al., 2005) or by FLP-out. In mice, the MADM system provides the means for clonal gene inactivation (Muzumdar et al., 2007).
Designing the stem cell hunt
The goal of lineage analysis as presented here is to identify large numbers of stem cell clones that are all derived from a particular type of stem cell. This requires that they be distinguished from transient clones in the tissue of interest. Once a sufficient pool of such clones becomes available, detailed study of clone anatomy and behavior will reveal the location and behavior of this type of stem cell. To efficiently detect stem cell clones one must first address a series of issues based on general knowledge of the tissue in question. A crucial parameter is the estimated turnover rate of the cells maintained by the candidate stem cells. For example, in the Drosophila ovary, one would determine the cell loss rate due to egg production and add the cell loss rate due to apoptosis. For many tissues these calculations will be crude estimates. Do them anyway. Even an order of magnitude estimate would likely distinguish a few rare clones generated by background recombination, from the much more frequent clones that would be produced by regular tissue renewal.
Experimental conditions
Stem cell behavior is strongly influenced by environmental conditions (Drummond-Barbosa and Spradling, 2001) so it is critically important to raise animals in such a manner that stem cell activity occurs uniformly and at high rates. For the Drosophila female germline stem cell, this entails using animals at least 4 days after eclosion that have been provided daily with fresh yeast paste, moisture, males, oviposition sites and appropriate odors to stimulate uniform, continuous egg production. However, the treatment required may differ for each tissue and stem cell, and will likely have to be optimized by trial and error at the same time stem cells are being sought.
Background
Background (non-induced) recombination is known to occur in most cell marking systems, generating cell clones whose time of origin differs from expectation. Such background effects should be measured and minimized, and any remaining background clones should be taken into account when analyzing clonal data.
When to induce clones
Differentiating between short-lived transient clones (see Movie 1) and long-lived stem cell clones (see Movie 2) is the key step in identifying stem cells. Hence the time elapsed between clone initiation and tissue analysis is the key variable. At short intervals there are likely to still be many transient clones, while very long intervals may reduce the number of single stem cell clones as described below. Clones should be initiated when adult tissue renewal is operating at steady state, which may not be the case in very young or old tissues. For example, many Drosophila tissues do not fully complete adult development until two days after eclosion, so it is unwise to use adults younger than 4 days in a study of adult stem cell behavior. Drosophila ovarian cap cells continue to divide during the first day or two after eclosion, but then become post-mitotic for the rest of adulthood (Ward et al., 2006; Song et al., 2007). Very old animals should not be used (at least initially) because stem cell and niche function often declines sharply in old age (Boyle et al., 2007; Pan et al., 2007; Wallenfang et al., 2006).
How many clones to induce
Another key consideration is the frequency of clone induction. Ideally, each clone would be entirely separate, and not touch any other labeled cells. Adjacent clones are likely to be scored incorrectly as a single clone, and too high a frequency of such errors may prove fatal. However, too low a frequency of clone labeling makes data acquisition slow and tedious. Experimenting with heat shock or tamoxifen levels allows the optimal clone frequency to be determined. The frequency of adjacent clones should be estimated and corrections applied.
When to examine clones
Understanding the dynamics of tissue renewal requires that stem cell clones be examined at many points in their growth. At least a few time points should be early, when both transient and stem cell clones co-exist, while slightly later time points are valuable to ensure that predominantly young stem cell clones are examined. Very long time points may be less useful because many stem cell clones will likely have turned over, while some of those remaining may have been altered by stem cell replacement (see section 4.2.4). For example, in the Drosophila midgut, transient clones are present only during the first 7 days after heat shock (Ohlstein and Spradling, 2006). Consequently, one would want to examine points before 7 days and to also sample clones between 7 and 21 days.
Identifying stem cell clones and stem cells
Once all the factors discussed above have been optimized, and extensive clonal data has been collected, one is finally in a position to analyze the results. Identifying stem cell clones and stem cells becomes a matter of applying the principles discussed earlier. At short periods after clone induction, transient clones will predominate, and will range in size from single cells to large clones, depending on where in the cell lineage the clone was induced and the number of transient divisions the lineage undergoes (see movies 1 and 5). Transient clones are not expected to contain any specific tissue region in common. Be aware that marked clones present in an actual tissue often appear more complex than in simple diagrams (see movies 1 and 2). Real clones may be irregular in shape due to cell migration (see movies 5 and 6 for animations of transient and follicle stem cell clones within the Drosophila ovariole).
Eventually, after the pre-existing tissue cells and transient clones have turned over, mostly stem cell clones will be present. In contrast to transient clones, stem cell clones are likely to be uniform in size as long as all stem cells are equally active (see Xie and Spradling, 1998). While it remains theoretically possible that a population of stem cells occupies a wide variety of surroundings, this situation has yet to be documented by lineage analysis in a metazoan tissue. Instead, a key feature of characterized stem cell clones is that they display an invariant feature that relates to the tissue architecture. This feature corresponds to the origins of the clones and provides information on the location of the stem cells and the niches that maintains them. For example, Drosophila germline stem cell clones always contain a labeled cell at the anterior of the ovariole, because this is where the stem cells reside (see movies 2 and 6). Drosophila intestinal stem cell clones always contain a cell adjacent to the basement membrane and within a diploid cell nest (Ohlstein and Spradling, 2006). Identifying the invariant feature that unites a homogeneous collection of stem cell clones represents the key insight that allows individual stem cells to be identified.
Once the location of the candidate stem cell is known, it should be possible to identify and follow the growth of young stem cell clones at the early time points when transient clones are more numerous. Clones with the appearance of transient clones at late time points should be explicable as resulting from stem cell loss (movie 3). Additionally, the apparent size of a stem clone may increase (often it will double) over time if one of the labeled stem cell's daughters replaces a second nearby stem cell, takes up residence in its niche, and spawns a second clone. This sequence of events will convert a single labeled stem cell clone into two adjacent marked stem cell clones that appear as one (movie 4). Replacement events may cause the average size of stem cell clones to increase with time, even after all transient clones have been lost. Once understood, the time sequence of clonal patterns in the tissue should become highly predictable. However, it is wise to verify one's conclusions by repeating the analysis with at least one independent lineage marking system.
Movie 3Stem cell replacement
In this hypothetical tissue, four stem cells (highlighted in dark blue at the beginning of the movie) are maintained in a niche (blue gradient highlighted in light blue at the beginning of the movie) produced by a population of non-dividing niche-generating cells (purple, highlighted in red at the beginning of the movie). Each stem cell daughter undergoes three transient divisions. Here, the daughter of an unmarked stem cell is shown to invade the niche of a neighboring marked stem cell and displace it. Stem cell replacement events can cause a decrease (movie 3), an increase (movie 4, replacement event 1), or no change (movie 4, replacement event 2) in stem cell clone size, depending on the label status of the lost and replacement cells.
Movie 4Stem cell replacement
In this hypothetical tissue, four stem cells (highlighted in dark blue at the beginning of the movie) are maintained in a niche (blue gradient highlighted in light blue at the beginning of the movie) produced by a population of non-dividing niche-generating cells (purple, highlighted in red at the beginning of the movie). Each stem cell daughter undergoes three transient divisions. Here, the daughter of an marked stem cell is shown to invade the niche of a neighboring unmarked stem cell and displace it. Stem cell replacement events can cause a decrease (movie 3), an increase (movie 4, replacement event 1), or no change (movie 4, replacement event 2) in stem cell clone size, depending on the label status of the lost and replacement cells.
There are two major reasons why it may still be difficult to rationalize the patterns of clonal labeling as described above. In looking for their “invariant feature” clones must be analyzed based on the anatomy and cell types that are known. However, many current descriptions of tissues are not accurate to single cell resolution. Actual tissues may contain more cell types, exhibiting more dynamic, environmentally influenced behavior, all within a three dimensional architecture more complex than what is recorded in histology textbooks. In such cases, it may first be necessary to more accurately describe the anatomy of a tissue, in order to understand clonal behavior and to identify stem cells.
Finally, one must also consider the possibility that self-renewing stem cells do not exist. Tissue renewal may take place by division of “differentiated” cells, or a uniform population of cells may replenish lost neighbors by dividing or differentiating at appropriate frequencies. The clones produced when such a tissue is subject to lineage analysis will depend on the detailed mechanisms involved. In general, maintenance via dedifferentiation will yield mostly small clones without a special tissue feature. Maintenance by a uniform progenitor population will generate clones that grow in size but decrease in number with time, much like stem cell clones from a population of stem cells that turn over and undergo replacement. These mechanisms can be distinguished by identifying young stem cell clones (to minimize replacement effects) and determining if they are consistently associated with a restricted tissue feature (the niche?).
Analyzing stem cells
Finding the stem cell niche
The stem cell niche is a specialized microenvironment that is required to maintain stem cell self-renewal. Some niches, such as those at the tip of the Drosophila ovariole and testis, are maintained by a population of fixed, non-dividing cells, while others, such as the niches supporting Drosophila follicle cell production, depend on dynamic cell populations and non-cellular factors such as extracellular matrices (Morrison and Spradling, 2008). Either way, niches will reside in non-random positions in the tissue. Once this has been established, they must be shown to function independently of their current stem cell residents. A true niche must persist after its current stem cells have been depleted and have the ability to maintain a newly introduced (competent) cell as a stem cell. The stem cell depletion required to address this question can be achieved through normal stem cell turnover or by genetically or pharmacologically inducing stem cell loss. If following such depletion new cells take up residence and become stem cells, then the existence of a niche can be confirmed.
Many other unanswered questions about niche function can be addressed using lineage methods. In particular, the ability of stem cell daughters to fill an available niche, as followed by lineage analysis, provides an ongoing assay for the location, genetic regulation and stability of niches in vivo. The niches within the Drosophila ovary and testis are always full, but it should be possible to detect stem cell movement from niche to niche over time in a tissue with empty niches (Xie and Spradling, 2000; Ryu et al., 2003).
Quantitative features of the stem cell lineage
Stem cell number
One of the fundamental questions in any stem cell-based tissue is the number of stem cells that are present. Assuming that all stem cells are equally active, stem cell clones provide an accurate estimate of this number. The fraction of tissue labeled by a single marked stem cell represents the inverse of the total number of stem cells. For example, in the Drosophila follicle stem cell lineage, follicle stem cell clones always comprise approximately 50% of the total follicle cell population of the ovariole (see Movie 6). It follows that each ovariole contains two (0.5−1) stem cells (Margolis and Spradling, 1995). In the Drosophila midgut, individual stem cell clones support about 15–20 total cells. Dividing the total number of cells by this figure yields an estimate of 800–1,000 intestinal stem cells in the posterior midgut (Ohlstein and Spradling, 2006).
Movie 5Follicle cell transient clone development
In the Drosophila ovary, two follicle stem cells (FSCs) reside in separate niches on opposite sides of the germarium. Daughters from each stem cell commingle and undergo several transient divisions to cover germline cysts. Despite these complexities, lineage analysis can be used to identify the stem cells and track clonally related cells over time. Here, the growth of a transient clone derived from the earliest non-stem cell daughter of the FSC lineage is shown. These transient clones can easily be distinguished from stem cell clones (movie 6) by the fact that the region containing labeled cells moves posteriorly with time and is soon lost.
Transient division rate
If a low enough clone frequency can be achieved to ensure that each transient clone is derived from a single recombination event, the maximum number of cells in a transient clone is related to the minimum number of transient divisions by the following equation:

Stem cell half-life
Stem cell turnover appears to be a simple concept, but in reality one must distinguish between a variety of distinct processes. Stem cell turnover most simply would represent the loss of a stem cell from its niche, a process that we refer to as “stem cell loss.” The standard equation for determining half-life (t1/2) in a system with exponential decay would be applied:
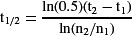
Stem cell replacement
Replacement is the loss of a stem cell from its niche and the substitution (“replacement”) of another cell which takes over stem cell function. In Drosophila, such events are surprisingly common (Margolis and Spradling, 1995; Xie and Spradling, 1998; Jin and Xie, 2007; Song and Xie, 2002; Song and Xie, 2003; Song et al., 2002; Movies 3 and 4). It appears likely that the incoming cell often actually “evicts” the resident stem cell and, thus, that stem cells within niches frequently engage in competition with external cells to maintain niche residency. In fact, stem cell loss without replacement has not been observed, except under conditions where the structure of the niche has become aberrant. This process we refer to as “niche loss.” In aging Drosophila gonads, the number of stem cells and niches declines slowly over time, indicating that niche loss does occur (Pan et al., 2007). However, it is much slower than the rate of stem cell replacement in this tissue.
Qualitative features of a stem cell lineage
Stem cell potency
Stem cell potency is defined as the total number of different cell types produced by a single type of stem cell. Such determinations are limited by the lack of a precise definition of “cell type.” One can never test all the conditions, whether natural or artificial, that might elicit an additional type of cell to be produced. Thus, potency cannot be definitively determined and always represents a minimum estimate.
Daughter cell migrations
Lineage analysis can provide detailed information about the movement of cells downstream of the stem cell. If daughter cells migrate away from the niche along stereotypical migration paths, this will be manifest as reproducible, progressive changes in the shapes of labeled clones over time. Analysis of bulk cell movement can be achieved, even when daughter cells migrate over long distances, undergo transient divisions, and intermingle extensively with cells from unrelated lineages. For example, Levy et al. found that following wounding of the mouse epidermis, labeled stem cell daughters in nearby hair follicles migrate upward and into the surrounding interfollicular epithelium (IEF), where they remain temporarily, but do not contribute to the long term renewal of the IEF (Levy et al., 2007).
Understanding cell migration at the single cell level is also possible, though the complexity rises exponentially with each transient division downstream from the stem cell. Thus, these studies are most effective for the characterization of cell movement in the earliest part of the lineage. For example, studies of the follicle stem cell lineage found that each daughter cell migrates away from the niche along one of two distinct migration paths in roughly alternating succession ((Nystul and Spradling, 2007), see Movie 6). The migration patterns of these early follicle cells were uncovered by observing very small stem cell clones, containing a single stem cell and only one or two daughter cells. This type of analysis requires that the stem cell be identifiable through means other than the clonal marking system being used to follow daughter cell migrations, so that transient clones still present in the tissue can be distinguished from stem cell clones.
Movie 6Follicle stem cell clone
In the Drosophila ovary, two follicle stem cells (FSCs) reside in separate niches on opposite sides of the germarium. Daughters from each stem cell commingle and undergo several transient divisions to cover germline cysts. Despite these complexities, lineage analysis can be used to identify the stem cells and track clonally related cells over time. Here, the growth of a follicle stem cell clone (red) is shown. Note that because only one of the two FSCs acquired the label in this example, once all transient cells have washed out, 50% of the follicle cell population is labeled. In addition, it is possible to identify which follicle cells were derived from each FSC.
Conclusion
Cell lineage studies have been used for more than one hundred years to address fundamental questions of embryonic development and differentiation. Today, in model organisms such as Drosophila and mouse, genetic constructs are available that greatly facilitate the ability to follow the division, growth, migration and differentiation of cells in tissues. These techniques make it much easier than in the past to identify new tissue stem cells, and to identify previously described stem cells with single-cell resolution. These new methods are stimulating rapid advances in stem cell biology, but the amount of work remaining is immense. Collecting three dimensional lineage data at single cell resolution in diverse tissues, and inverting the resulting data sets to reveal stem cell number, location and behavior remains a slow process. Developing and implementing automated microscopes and software with the capacity to speed up this process represents a potentially fruitful area of stem cell research that has received far too little attention (Keller et al., 2008).
Acknowledgments
The authors thank Rafael Villagaray (Carnegie Institution) and Andrea Stevenson Won (Biomodal) for artistry and development of the movies, and Rebecca Frederick and Tina Tootle (Carnegie Institution) for helpful comments on the manuscript.
References
- Whitman C. The embryology of Clepsine. Q. J. Microscop. Sci (N.S.). 1898;18:215–315.
- Stent G.S, Weisblat D.A. Cell lineage in the development of invertebrate nervous systems. Annu Rev Neurosci. 1985;8:45–70. [PubMed: 3885830] [CrossRef]
- Sulston J.E, et al. The embryonic cell lineage of the nematode Caenorhabditis elegans. Dev Biol. 1983;100(1):64–119. [PubMed: 6684600] [CrossRef]
- Chalfie M, Horvitz H.R, Sulston J.E. Mutations that lead to reiterations in the cell lineages of C. elegans. Cell. 1981;24(1):59–69. [PubMed: 7237544] [CrossRef]
- Vogt W. Sitzungsber. Ges. Morph. Physiol. Munchen. 1924;35:22–32.
- Axelrod D. Carbocyanine dye orientation in red cell membrane studied by microscopic fluorescence polarization. Biophys J. 1979;26(3):557–573. [PMC free article: PMC1328570] [PubMed: 263688] [CrossRef]
- Rosenquist G. A radioautographic study of labeled grafts in the chick blastoderm. Development from primitive-streak stages to stage 12II. Studies on the mechanics of notochord elongation and somite formation. Contrib. Embryol. Carnegie Inst. Wash. 1966;38:71–110.
- Lawson K.M, JJ Pedersen R.A. Cell fate and cell lineage in the endoderm of the presomite mouse embryo, studied with an intracellular tracer. Dev Biol. 1986;115:325–339. [PubMed: 3709966] [CrossRef]
- Price J.T, D Cepko C. Lineage analysis in the vertebrate nervous system by retrovirus-mediated gene transfter. PNAS. 1987;84:156–160. [PMC free article: PMC304161] [PubMed: 3099292] [CrossRef]
- Lemischka I.R, Raulet D.H, Mulligan R.C. Developmental potential and dynamic behavior of hematopoietic stem cells. Cell. 1986;45(6):917–927. [PubMed: 2871944] [CrossRef]
- Jordan C.T, Lemischka I.R. Clonal and systemic analysis of long-term hematopoiesis in the mouse. Genes Dev. 1990;4(2):220–232. [PubMed: 1970972] [CrossRef]
- Engelhardt J.F, et al. Progenitor cells of the adult human airway involved in submucosal gland development. Development. 1995;121(7):2031–2046. [PubMed: 7635050]
- Golden J.A, Fields-Berry S.C, Cepko C.L. Construction and characterization of a highly complex retroviral library for lineage analysis. Proc Natl Acad Sci USA. 1995;92(12):5704–5708. [PMC free article: PMC41765] [PubMed: 7777573] [CrossRef]
- Sturtevant A. The claret mutant type of Drosophila simulans: A study of chromosome elimination and cell lineage. Z Wiss Zool. 1929;135:325–356.
- Garcia-Bellido A, Merriam J.R. Cell lineage of the imaginal discs in Drosophila gynandromorphs. J Exp Zool. 1969;170(1):61–75. [PubMed: 5780530] [CrossRef]
- Collinson J.M, et al. Clonal analysis of patterns of growth, stem cell activity, and cell movement during the development and maintenance of the murine corneal epithelium. Dev Dyn. 2002;224(4):432–440. [PubMed: 12203735] [CrossRef]
- Cohnheim V. Congenitales, quergestreiftes Muskelsarkom der Nieren. Virchows Archiv fur Pathologische Anatomie und Physiologie und fur Klinische Medizin. 1875;65:64–69. [CrossRef]
- Garcia S.B, Novelli M, Wright N.A. The clonal origin and clonal evolution of epithelial tumours. Int J Exp Pathol. 2000;81(2):89–116. [PMC free article: PMC2517717] [PubMed: 10762440] [CrossRef]
- Moore M.A, Owen J.J. Chromosome marker studies on the development of the haemopoietic system in the chick embryo. Nature. 1965;208(5014):956 passim. [PubMed: 5868856] [CrossRef]
- Le Douarin N, Barq G. Use of Japanese quail cells as “biological markers” in experimental embryology. C R Acad Sci Hebd Seances Acad Sci D. 1969;269(16):1543–1546. [PubMed: 4989896]
- Abramson S. The identification in adult bone marrow of plutipotent and restricted stem cells of the myeloid and lymphoid systems. J. Exp. Med. 1979;145:1567–1579. [PMC free article: PMC2180675] [PubMed: 140917] [CrossRef]
- Ueno H, Weissman I.L. Clonal analysis of mouse development reveals a polyclonal origin for yolk sac blood islands. Dev Cell. 2006;11(4):519–533. [PubMed: 17011491] [CrossRef]
- Stern C. Genetic Mosaics. Vol. 251. Cambridge: Harvard University Press; 1968. p. 68.
- Garcia-Bellido A, Merriam J.R. Genetic analysis of cell heredity in imaginal discs of Drosophila melanogaster. Proc Natl Acad Sci USA. 1971;68(9):2222–2226. [PMC free article: PMC389389] [PubMed: 5002430] [CrossRef]
- Wieschaus E, Szabad J. The development and function of the female germ line in Drosophila melanogaster: a cell lineage study. Dev Biol. 1979;68(1):29–46. [PubMed: 108155] [CrossRef]
- Golic K.G. Site-specific recombination between homologous chromosomes in Drosophila. Science. 1991;252(5008):958–961. [PubMed: 2035025] [CrossRef]
- Lakso M, et al. Targeted oncogene activation by site-specific recombination in transgenic mice. Proc Natl Acad Sci USA. 1992;89(14):6232–6236. [PMC free article: PMC49474] [PubMed: 1631115] [CrossRef]
- Golic K.G, Golic M.M. Engineering the Drosophila genome: chromosome rearrangements by design. Genetics. 1996;144(4):1693–1711. [PMC free article: PMC1207720] [PubMed: 8978056]
- Duffy J.B, Harrison D.A, Perrimon N. Identifying loci required for follicular patterning using directed mosaics. Development. 1998;125(12):2263–2271. [PubMed: 9584125]
- Harrison D.A, Perrimon N. Simple and efficient generation of marked clones in Drosophila. Curr Biol. 1993;3(7):424–433. [PubMed: 15335709] [CrossRef]
- Margolis J, Spradling A. Identification and behavior of epithelial stem cells in the Drosophila ovary. Development. 1995;121(11):3797–3807. [PubMed: 8582289]
- Decotto E, Spradling A.C. The Drosophila ovarian and testis stem cell niches: similar somatic stem cells and signals. Dev Cell. 2005;9(4):501–510. [PubMed: 16198292] [CrossRef]
- Ohlstein B, Spradling A. The adult Drosophila posterior midgut is maintained by pluripotent stem cells. Nature. 2006;439(7075):470–474. [PubMed: 16340960] [CrossRef]
- Struhl G, Basler K. Organizing activity of wingless protein in Drosophila. Cell. 1993;72(4):527–540. [PubMed: 8440019] [CrossRef]
- Merkle F.T, Mirzadeh Z, Alvarez-Buylla A. Mosaic organization of neural stem cells in the adult brain. Science. 2007;317(5836):381–384. [PubMed: 17615304] [CrossRef]
- Brand A.H, Perrimon N. Targeted gene expression as a means of altering cell fates and generating dominant phenotypes. Development. 1993;118(2):401–415. [PubMed: 8223268]
- Han D.D, Stein D, Stevens L.M. Investigating the function of follicular subpopulations during Drosophila oogenesis through hormone-dependent enhancer-targeted cell ablation. Development. 2000;127(3):573–583. [PubMed: 10631178]
- Nicholson L, et al. Spatial and temporal control of gene expression in Drosophila using the inducible GeneSwitch GAL4 system. I. Screen for larval nervous system drivers. Genetics. 2008;178(1):215–234. [PMC free article: PMC2206072] [PubMed: 18202369] [CrossRef]
- Webster N.J, et al. The hormone-binding domains of the estrogen and glucocorticoid receptors contain an inducible transcription activation function. Cell. 1988;54(2):199–207. [PubMed: 3390864] [CrossRef]
- Forde A, et al. Temporal Cre-mediated recombination exclusively in endothelial cells using Tie2 regulatory elements. Genesis. 2002;33(4):191–197. [PubMed: 12203917] [CrossRef]
- Osterwalder T, et al. A conditional tissue-specific transgene expression system using inducible GAL4. Proc Natl Acad Sci USA. 2001;98(22):12596–12601. [PMC free article: PMC60099] [PubMed: 11675495] [CrossRef]
- Roman G, et al. P[Switch], a system for spatial and temporal control of gene expression in Drosophila melanogaster. Proc Natl Acad Sci USA. 2001;98(22):12602–12607. [PMC free article: PMC60100] [PubMed: 11675496] [CrossRef]
- Nakagawa T, Nabeshima Y, Yoshida S. Functional identification of the actual and potential stem cell compartments in mouse spermatogenesis. Dev Cell. 2007;12(2):195–206. [PubMed: 17276338] [CrossRef]
- Clayton E, et al. A single type of progenitor cell maintains normal epidermis. Nature. 2007;446(7132):185–189. [PubMed: 17330052] [CrossRef]
- Barker N, et al. Identification of stem cells in small intestine and colon by marker gene Lgr5. Nature. 2007;449(7165):1003–1007. [PubMed: 17934449] [CrossRef]
- Hunter N.L, et al. Ligand-activated Flpe for temporally regulated gene modifications. Genesis. 2005;41(3):99–109. [PubMed: 15729687] [CrossRef]
- Heidmann D, Lehner C.F. Reduction of Cre recombinase toxicity in proliferating Drosophila cells by estrogen-dependent activity regulation. Dev Genes Evol. 2001;211(8–9):458–465. [PubMed: 11685583] [CrossRef]
- Siegal M.L, Hartl D.L. Transgene Coplacement and high efficiency site-specific recombination with the Cre/loxP system in Drosophila. Genetics. 1996;144(2):715–726. [PMC free article: PMC1207562] [PubMed: 8889532]
- Nystul T, Spradling A. An epithelial niche in the Drosophila ovary undergoes long-range stem cell replacement. Cell Stem Cell. 2007;1(3):277–285. [PubMed: 18371362] [CrossRef]
- Zong H, et al. Mosaic analysis with double markers in mice. Cell. 2005;121(3):479–492. [PubMed: 15882628] [CrossRef]
- Dang D.T, Perrimon N. Use of a yeast site-specific recombinase to generate embryonic mosaics in Drosophila. Dev Genet. 1992;13(5):367–375. [PubMed: 1292893] [CrossRef]
- Xu T, Rubin G.M. Analysis of genetic mosaics in developing and adult Drosophila tissues. Development. 1993;117(4):1223–1237. [PubMed: 8404527]
- Lee T, Luo L. Mosaic analysis with a repressible cell marker (MARCM) for Drosophila neural development. Trends Neurosci. 2001;24(5):251–254. [PubMed: 11311363] [CrossRef]
- Ma J, Ptashne M. The carboxy-terminal 30 amino acids of GAL4 are recognized by GAL80. Cell. 1987;50(1):137–142. [PubMed: 3297349] [CrossRef]
- Kirilly D, et al. BMP signaling is required for controlling somatic stem cell self-renewal in the Drosophila ovary. Dev Cell. 2005;9(5):651–662. [PubMed: 16256740] [CrossRef]
- Muzumdar M.D, Luo L, Zong H. Modeling sporadic loss of heterozygosity in mice by using mosaic analysis with double markers (MADM). Proc Natl Acad Sci USA. 2007;104(11):4495–4500. [PMC free article: PMC1810340] [PubMed: 17360552] [CrossRef]
- Drummond-Barbosa D, Spradling A.C. Stem cells and their progeny respond to nutritional changes during Drosophila oogenesis. Dev Biol. 2001;231(1):265–278. [PubMed: 11180967] [CrossRef]
- Ward E.J, et al. Stem cells signal to the niche through the Notch pathway in the Drosophila ovary. Curr Biol. 2006;16(23):2352–2358. [PubMed: 17070683] [CrossRef]
- Song X, et al. Notch signaling controls germline stem cell niche formation in the Drosophila ovary. Development. 2007;134(6):1071–1080. [PubMed: 17287246] [CrossRef]
- Boyle M, et al. Decline in self-renewal factors contributes to aging of the stem cell niche in the Drosophila testis. Cell Stem Cell. 2007;1(4):470–478. [PubMed: 18371382] [CrossRef]
- Pan L, et al. Stem cell aging is controlled both intrinsically and extrinsically in the Drosophila ovary. Cell Stem Cell. 2007;1(4):458–469. [PubMed: 18371381] [CrossRef]
- Wallenfang M.R, Nayak R, DiNardo S. Dynamics of the male germline stem cell population during aging of Drosophila melanogaster. Aging Cell. 2006;5(4):297–304. [PubMed: 16800845] [CrossRef]
- Xie T, Spradling A.C. Decapentaplegic is essential for the maintenance and division of germline stem cells in the Drosophila ovary. Cell. 1998;94(2):251–260. [PubMed: 9695953] [CrossRef]
- Morrison S.J, Spradling A.C. Stem cells and niches: mechanisms that promote stem cell maintenance throughout life. Cell. 2008;132(4):598–611. [PMC free article: PMC4505728] [PubMed: 18295578] [CrossRef]
- Xie T, Spradling A.C. A niche maintaining germ line stem cells in the Drosophila ovary. Science. 2000;290(5490):328–330. [PubMed: 11030649] [CrossRef]
- Ryu B.Y, et al. Stem cell and niche development in the postnatal rat testis. Dev Biol. 2003;263(2):253–263. [PubMed: 14597200] [CrossRef]
- Jin Z, Xie T. Dcr-1 maintains Drosophila ovarian stem cells. Curr Biol. 2007;17(6):539–44. [PubMed: 17306537] [CrossRef]
- Song X, Xie T. DE-cadherin-mediated cell adhesion is essential for maintaining somatic stem cells in the Drosophila ovary. Proc Natl Acad Sci USA. 2002;99(23):14813–14818. [PMC free article: PMC137501] [PubMed: 12393817] [CrossRef]
- Song X, Xie T. Wingless signaling regulates the maintenance of ovarian somatic stem cells in Drosophila. Development. 2003;130(14):3259–3268. [PubMed: 12783796] [CrossRef]
- Song X, et al. Germline stem cells anchored by adherens junctions in the Drosophila ovary niches. Science. 2002;296(5574):1855–1857. [PubMed: 12052957] [CrossRef]
- Levy V, et al. Epidermal stem cells arise from the hair follicle after wounding. Faseb J. 2007;21(7):1358–66. [PubMed: 17255473] [CrossRef]
- Orban P.C, Chui D, Marth J.D. Tissue- and site-specific DNA recombination in transgenic mice. Proc Natl Acad Sci USA. 1992;89(15):6861–6865. [PMC free article: PMC49604] [PubMed: 1495975] [CrossRef]
- Metzger D, et al. Conditional site-specific recombination in mammalian cells using a ligand-dependent chimeric Cre recombinase. Proc Natl Acad Sci USA. 1995;92(15):6991–6995. [PMC free article: PMC41457] [PubMed: 7624356] [CrossRef]
- Dymecki S.M. A modular set of Flp, FRT and lacZ fusion vectors for manipulating genes by site-specific recombination. Gene. 1996;171(2):197–201. [PubMed: 8666272] [CrossRef]
- Keller P.J, Schmidt A.D, Wittbrodt J, Stelzer E.H.K. Reconstruction of zebrafish early embryonic development by scanned light sheet microscopy. Science. 2008;322:1065–1069. [PubMed: 18845710] [CrossRef]
- *
Edited by Haifan Lin and Patricia Donahoe. Last revised November 25, 2008. Published January 31, 2009. This chapter should be cited as: Fox, D.T., Morris, L.X., Nystul, T., and Spradling, A.C., Lineage analysis of stem cells (January 31, 2009), StemBook, ed. The Stem Cell Research Community, StemBook, doi/10.3824/stembook.1.33.1, http://www.stembook.org.
- Review Neural crest lineage analysis: from past to future trajectory.[Development. 2020]Review Neural crest lineage analysis: from past to future trajectory.Tang W, Bronner ME. Development. 2020 Oct 23; 147(20). Epub 2020 Oct 23.
- Lineage tree for the venous pole of the heart: clonal analysis clarifies controversial genealogy based on genetic tracing.[Circ Res. 2012]Lineage tree for the venous pole of the heart: clonal analysis clarifies controversial genealogy based on genetic tracing.Lescroart F, Mohun T, Meilhac SM, Bennett M, Buckingham M. Circ Res. 2012 Oct 26; 111(10):1313-22. Epub 2012 Aug 1.
- Review [Application and Research Progress of Lineage Tracing in Differentiation Mechanism of Hematopoietic Stem Cells--Review].[Zhongguo Shi Yan Xue Ye Xue Za...]Review [Application and Research Progress of Lineage Tracing in Differentiation Mechanism of Hematopoietic Stem Cells--Review].Dong Y, Dong F, Bai HT, Ema H. Zhongguo Shi Yan Xue Ye Xue Za Zhi. 2021 Oct; 29(5):1690-1694.
- Review In-vivo differentiation of adult hematopoietic stem cells from a single-cell point of view.[Curr Opin Hematol. 2020]Review In-vivo differentiation of adult hematopoietic stem cells from a single-cell point of view.Nazaraliyev A, Richard E, Sawai CM. Curr Opin Hematol. 2020 Jul; 27(4):241-247.
- Review Dual recombinases-based genetic lineage tracing for stem cell research with enhanced precision.[Sci China Life Sci. 2021]Review Dual recombinases-based genetic lineage tracing for stem cell research with enhanced precision.Jin H, Liu K, Zhou B. Sci China Life Sci. 2021 Dec; 64(12):2060-2072. Epub 2021 Apr 9.
- Lineage analysis of stem cells - StemBookLineage analysis of stem cells - StemBook
- dbVar for Gene (Select 9829) (561)dbVar
- Homo sapiens rubicon like autophagy enhancer (RUBCNL), transcript variant 4, mRN...Homo sapiens rubicon like autophagy enhancer (RUBCNL), transcript variant 4, mRNAgi|1677499922|ref|NM_001286763.3|Nucleotide
- PMC Links for Gene (Select 18813) (36)PMC
- protein YIPF2 [Mus musculus]protein YIPF2 [Mus musculus]gi|326937439|ref|NP_001192086.1|Protein
Your browsing activity is empty.
Activity recording is turned off.
See more...