All rights reserved. The Regional Office for Europe of the World Health Organization welcomes requests for permission to reproduce or translate its publications, in part or in full. Address requests for publications of the WHO Regional Office for Europe to: Publications, WHO Regional Office for Europe, Scherfigsvej 8, DK-2100 Copenhagen Ø, Denmark. Alternatively, complete an online request form for documentation, health information, or for permission to quote or translate, on the Regional Office web site (http://www.euro.who.int/pubrequest).
NCBI Bookshelf. A service of the National Library of Medicine, National Institutes of Health.
WHO Guidelines for Indoor Air Quality: Selected Pollutants. Geneva: World Health Organization; 2010.
General description
Tetrachloroethylene (PCE) (CAS Registry Number 127-18-4; C2Cl4; molecular weight 165.83) is a readily volatile, colourless liquid with an ether-like smell (1). Its main physical and chemical properties are as follows (2–5): molecular weight 165.83 g/mol; density (at 20 °C) 1.6227 g/ml; melting point approximately −22 °C; boiling point 121 °C; water solubility (at 25 °C) 150 mg/litre; vapour pressure 18.47 mmHg at 25 °C (2), 1.9 kPa at 20 °C, 3.2 kPa at 30 °C and 6.0 kPa at 40 °C (3); Henry's Law constant 0.018 atm-m3/mol at 25 °C; log Kow (octanol/water partition coefficient) 3.40 (measurement value) and 2.97 (estimated value); and log Koc (octanol/carbon partition coefficient) 177∼350 (measurement value). The reaction rate constant against the hydroxyl radical is 1.7 × 10−13 cm3/molecule per second at 25 °C, against ozone 2.0 × 10−23 cm3/molecule per second at 25 °C and against the NO3 radical 5.2 × 10−17 cm3/molecule per second.
Its structural formula is:
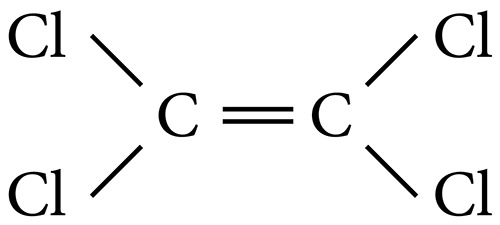
Common synonyms include perchloroethylene, PCE, ethylene tetrachloride, tetrachloroethene, 1,1,2,2-tetrachloroethylene, carbon dichloride, perchlor, tetrachloroethane, carbon bichloride, PERC, PCE and perk.
Conversion factors
At 760 mmHg and 20 °C, 1 ppm = 6.897 mg/m3 and 1 mg/m3 = 0.145 ppm; at 25 °C, 1 ppm = 6.782 mg/m3 and 1 mg/m3 = 0.147 ppm.
Applications and uses
Major industrial applications of PCE are as a synthetic raw material of hydrochlorofluorocarbon, a dry cleaning agent, a degreaser for manufactured metal parts and an industrial solvent. Other applications include the finishing and processing of textiles, the production of paint removers and printing inks, and the formulation of adhesives and specialized cleaning fluids. Consumer products that may contain PCE include adhesives, fragrances, spot removers, stain removers, fabric finishes, water repellents, wood cleaners, motor vehicle cleaners and dry-cleaned fabrics (6–8). In Japan, 69%, 18%, 12%, and 1.3% of PCE was used as a synthetic raw material of hydrochlorofluorocarbon, a dry cleaning agent, a degreaser and an industrial solvent, respectively (9).
Production
The estimated production volume in the United States in 1990 was 137 kilotonnes, compared to 282 kilotonnes in 1980 (2). In 1994, the total production of PCE in the EU was 164 kilotonnes. Of this amount, 78 kilotonnes was sold for use within the EU, 56 kilotonnes were exported and the remainder used as a chemical intermediate within the chemical industry (10). For Japan and the United States, it was 178 and 102 kilotonnes, respectively (1). In Canada, where PCE is no longer produced, the demand in 1990 was estimated at 14 kilotonnes (7). In Japan, the production and supply of PCE was 25 and 37 kilotonnes, respectively. No estimates of consumption levels are available for other parts of the world.
Analytical methods in air
Common methods of measuring indoor concentrations of TCE include integrated active and passive sampling methods using tubes packed with a carbon-based sorbent or evacuated SUMMA canisters (passivated canister sampling apparatus), or diffusion samplers such as charcoal badges. Canister sampling involves controlling the flow of air into a pre-evacuated canister. Sorbent tubes and badges retain compounds according to the affinity of the sorbent for that compound. For sorbent or charcoal sampling, the analytes must first be extracted thermally or chemically, then separated using gas chromatography and identified using a detection method such as mass spectrometry (11). Personal exposure studies often use these sorbent-based methods.
Environmental fate
There are no known natural sources of PCE. The Henry's Law constant indicates that PCE is expected to volatilize rapidly from water surfaces and that volatilization from moist soil surfaces may occur (1). Concentrations in ambient air may fluctuate widely over relatively short periods of time depending on the strength of the emission source, variations in wind direction and velocity, and scavenging and photodecomposition (6).
The half-life in the atmosphere has been estimated to range from 70 to 250 days (2).Considering the atmospheric concentrations and reaction rate constant of the hydroxyl radical and ozone, this removal from atmosphere is mostly due to reaction with hydroxyl radicals. This process is temperature-dependent, with increased reaction rates in the summer. The results of biodegradability tests suggested that the biodegradability of PCE is extremely low in environmental water (12,13); it may persist in groundwater for several months or more (7).
The main sources resulting in ambient air concentrations are industrial emissions and releases from building and consumer products. Releases are primarily to the atmosphere, but the compound is also released to surface water and land in sewage sludges and in other liquid and solid waste, where it can evaporate rapidly to the atmosphere.
ATSDR has estimated that 80–85% of the PCE used annually in the United States is released into the atmosphere (2). Atmospheric emissions result from evaporation during dry cleaning, metal degreasing, the production of fluorocarbons and other chemicals, and miscellaneous solvent-associated applications (14). However, the percentage is likely to be lower in the United States at present, owing to improved technology and regulatory restrictions. Almost all PCE enters the atmosphere unchanged. A small proportion enters water and wastewater. From surface water, PCE volatilizes owing to its relatively low water solubility and high vapour pressure.
Indoor sources and pathways of exposure
Inhalation is the most common route of exposure to PCE for the general population. Ingestion of contaminated drinking-water may also be important in areas with highly contaminated water. While PCE has been found in some food items, particularly from local source contamination, not enough data are available to estimate exposure through this route (2).
Indoor sources
Consumer products described above are sources of indoor PCE exposure. Contaminated drinking-water may be a source of indoor PCE exposure when taking a shower or washing dishes. Workers from dry cleaning establishments have been found to become sources of PCE at home by exhaling PCE in their breath (15). Dry-cleaned clothes are also possible sources of PCE in a house.
The air in dry cleaning facilities and neighbouring homes or facilities can contain elevated concentrations of PCE. Soil pollution with PCE has resulted in indoor air concentrations of these chemicals in residential homes. These chemicals can evaporate from the soil, permeate through floors and result in elevated indoor air concentrations. PCE can permeate through synthetic water pipes and contaminate drinking-water, thus leading to exposure during bathing or showering.
Drinking-water
Exposure can take place by drinking contaminated water as well as volatilization. In the United States, PCE is not detectable in drinking-water in most cases. The EPA Groundwater Supply Survey of 945 drinking-water systems nationwide reported PCE in 75 out of 945 systems in 1984; the median level of the positive samples was about 0.75 μg/litre, with a maximum of 69 μg/litre (2). In several studies in Canada, PCE was detectable in 6–60% of the tested samples. Reported mean values ranged from 0.1 to 0.9 μg/litre (6). In several other countries, including Germany and the United Kingdom, similar results have been observed (8,16). In some villages in Finland, concentrations of up to 180 μg/litre were found (17). In Japan, PCE levels in 99.7% of drinking-water (n = 5600) were less than 1 μg/litre with a maximum of 7 μg/litre in 2007 (18).
Food
Data on concentrations of PCE in food are scarce. In some studies from Germany and Switzerland reported in the early 1980s, relatively high total intakes of 87–170 μg/day were found (16). The results of market basket surveys in the United States, reported in 1987 and 1988, indicated lower levels. From the results of these surveys, total daily intake via food has been estimated at 0.12–65 μg/kg body weight (6). Several groups of researchers have reported elevated concentrations of PCE in fatty food products in residences and markets, owing to contamination from dry cleaning establishments nearby. In a supermarket near a dry cleaning shop in Germany, concentrations were 36 μg/kg and 110 μg/kg in cheese and margarine, respectively (8). In one instance in the United States, a very high PCE concentration was found in margarine (up to 50 mg/kg) in a shop next door to a dry cleaning establishment (2). Moreover, food grown on contaminated soil can contain PCE. In Japan, the daily maximum levels of PCE in the diet between 1990 and 1999 (n = 72∼81 in each year) were 4.4 μg/kg wet weight (14).
Indoor air concentrations and relationship with outdoor levels
Residential concentrations
In several United States cities (New York, Los Angeles, Chicago, Minneapolis, St Paul, Baltimore, Elizabeth and Houston), median indoor residential concentrations ranged from 0.4 μg/m3 in spring (Minneapolis) to 3.5 μg/m3 during winter (New York City) (19–26). The 90th percentiles ranged from 1 μg/m3 in Minneapolis in the spring to 14 μg/m3 in New York City during the summer (23).
Geometric mean concentrations of PCE in the homes of dry cleaning workers have been found to be 265 μg/m3, while non-exposed homes had a mean concentration of 2 μg/m3(15). Also, shops, offices or homes that share a building with a dry cleaning establishment have been found to have higher levels of PCE than those that are not near dry cleaners. A study of apartments in neighbourhoods with different income levels and ethnic compositions in New York City between 2001 and 2003 found overall geometric mean levels of 34 μg/m3, although in low-income neighbourhoods, the geometric mean was 256 μg/m3. Apartments with no dry cleaners in the building had a geometric mean concentration of 3 μg/m3. Before 1997, when New York adopted stricter regulations for dry cleaners, PCE was found to be about 340–360 μg/m3 in residential buildings with a dry cleaning establishment (27).
In a national survey of dwellings in France, a median concentration of 1.4 μg/m3 was found, with a 90th percentile of 5.2 μg/m3(28). In the European multinational urban air study EXPOLIS (29), median levels of PCE inside homes in Helsinki were not detectable, while they were 0.6 μg/m3 in Basel. The 90th percentile in Basel was 2.9 μg/m3. In Oxford, United Kingdom, the median indoor residential PCE concentration was 1.9 μg/m3, and 6.3 μg/m3 at the 90th percentile. Homes in Athens had a median indoor level of 4 μg/m3 and a 90th percentile level of 14.3 μg/m3. Milan and Prague were found to have median concentrations of 7.4 and 8.7 μg/m3, respectively, with 90th percentiles of 28.1 μg/m3 and 26.1 μg/m3, respectively. Median outdoor residential PCE levels were under the detection limit in Helsinki, 0.7 μg/m3 in Basel and 1.7 μg/m3 in Oxford. The 90th percentiles in Basel and Oxford were 1.4 μg/m3 and 3.4 μg/m3, respectively. In Athens, Milan and Prague, the median outdoor residential levels were 2.3 μg/m3, 4.1 μg/m3 and 5.3 μg/m3, respectively. Median personal exposure levels were lower than the indoor levels.
In 25 homes surveyed in Shimizu, Japan, geometric mean indoor PCE concentrations were 0.16 μg/m3 in summer and winter, with geometric standard deviations of 2.34 μg/m3 in summer and 2.77 μg/m3 in winter (30). The geometric mean concentration outside these homes was 0.11 μg/m3 in both summer and winter, with geometric standard deviations of approximately 2 μg/m3 in both seasons. In a national survey of dwellings in Japan, median indoor PCE concentrations in residential houses in 1997 (n = 180) and 1998 (n = 205) were 0.4 μg/m3 (mean 1.8 μg/m3; maximum 83.5 μg/m3) and 0.3 μg/m3 (mean 1.9 μg/m3; maximum 43.4 μg/m3), respectively (31).
Non-residential microenvironments
Predicted PCE concentrations in the air around metal degreasing machines were found to range from about 10 000 μg/m3 to above 1 million μg/m3, depending on the type of machine. In Germany, mean personal air samples in occupational environments with degreasers ranged from 18 000 to 40 000 μg/m3(10).
Exposure to PCE from dry cleaning affects both workers and members of the general public. A study in Finland of personal exposure of workers in commercial and industrial dry cleaning operations using dry-to-dry machines found that machine operators had the highest exposure levels (28 000 and 32 000 μg/m3 at commercial and industrial sites, respectively). Customer service workers had the lowest exposures, with an average of about 800 μg/m3(32). Another study of dry cleaning establishments in Chicago found indoor levels of PCE ranging from 12 000 to 355 000 μg/m3(33).
A survey of stores in a one-level commercial building in New Jersey found a median concentration of 690 μg/m3 and a 90th percentile of 4200 μg/m3 in a dry cleaning establishment in the building, and a median of 570 μg/m3 and 90th percentile of 5800 μg/m3 (8 hour average) in a neighbouring clothes rental shop, which may have also contained dry-cleaned clothes (34). The neighbouring shop on the other side had lower levels, but the median was still nearly 90 μg/m3, a level much higher than the average indoor environment. Dry cleaned clothes have been shown to emit PCE (2,32).
A survey of 70 office buildings across the United States (in which there were no complaints) found a median indoor PCE level of 1.5 μg/m3 and a 95th percentile of 18 μg/m3(35). A study of mechanically ventilated non-office and office buildings in Hong Kong SAR, China found arithmetic mean levels of 1.4 μg/m3 (SD = 3.4) and 1.9 μg/m3 (SD = 9.2), respectively (36). Median indoor concentrations at workplaces of the EXPOLIS participants were under the detection limit in Helsinki, 1.2 μg/m3 in Basel, 3.5 μg/m3 in Athens, 5.4 μg/m3 in Milan and 3.7 μg/m3 in Prague. The 90th percentile of workplace levels were 3.1 μg/m3 in Basel, 10.6 μg/m3 in Athens, 17.5 μg/m3 in Milan and 10 μg/m3 in Prague. The workplaces were typically offices. A survey of stores in Boston, MA found that geometric mean levels of PCE in stores and restaurants were near or under 3 μg/m3(37).
Ambient air
Residential outdoor median concentrations ranged from 0.3 μg/m3 in Minneapolis (spring) to 1.7 μg/m3 in Los Angeles (winter). The median outdoor concentrations of PCE in Shimizu (Japan) were 0.2 μg/m3 (mean 0.5 μg/m3; maximum 4.7 μg/m3) and 0.2 μg/m3 (mean 0.7 μg/m3; maximum 10.8 μg/m3), respectively (30). Outdoor levels in rural Canada were found to have a mean of 0.04 μg/m3 and a maximum of 7 μg/m3(38).
Indoor : outdoor ratios in New York and Los Angeles from a study in a low-income group of teenagers were between 1 and 2 at the median and did not have large standard deviations relative to the median (except for New York homes in the winter) (23). The earlier Total Exposure Assessment Methodology (TEAM) study found higher indoor : outdoor ratios in the cities of Bayonne and Elizabeth, NJ, with arithmetic means ranging from 2 in summer to 7 in winter and 5 in the winter in Los Angeles (39,40). The medians were not reported but are likely to have been lower than the arithmetic means.
Personal exposure
Median personal exposures of the teenagers were 2.2 μg/m3 in Los Angeles and 3.7 μg/m3 in New York, although the mean in New York was 8.8 μg/m3, indicating a more skewed distribution with some higher values (19–26). The New York indoor home distribution was also fairly skewed. The RIOPA study in Los Angeles, Elizabeth and Houston found that adult personal air concentrations had lower medians than means, with a particularly large difference in Elizabeth, where the median was 0.56 μg/m3 and the mean was 17.3 μg/m3(26). Indoor and outdoor residential mean concentrations in Elizabeth, however, were near 1 μg/m3 and were detectable in only 24% of outdoor samples and 39% of indoor samples, while in personal samples PCE was detected in 44% of the samples (26).
Personal exposures to PCE are most influenced by indoor concentrations. PCE can reach extremely high levels indoors, even in non-occupational environments such as homes or businesses that are in the same building as or close to dry cleaners. The TEAM studies found that higher personal PCE exposures were associated with visits to dry cleaners (39,40). Unlike other industries that use PCE, such as metal degreasing, dry cleaners are often located in residential, non-industrial commercial and business districts and may affect both the occupants of neighbouring establishments and food. Indoor environments next to dry cleaners have been found to have PCE concentrations of several hundreds of μg/m3. Indoor environments without sources of PCE have concentrations well below 10 μg/m3, with median concentrations near 1 μg/m3 in many areas. Dry-cleaned items can be an occasional source of PCE in some homes.
Toxicokinetics
Absorption
PCE is readily absorbed in the gastrointestinal tract and the lungs. Pulmonary uptake is proportional to ventilation rate, duration of exposure and, at lower atmospheric concentrations, the concentration in the inspired air (2). In both humans and animals, initial uptake following inhalation is rapid, with rates levelling off after a few hours of exposure. The available data suggest that a high proportion is absorbed in humans, but actual percentages have not been reported. In rats, the proportion absorbed was approximately 55–70% after 1 minute, gradually declining to 40–50% after 2 hours (41).
Dermal absorption from the gaseous phase is negligible. However, dermal absorption of significant amounts of PCE is probably possible in some situations. Dermal absorption of PCE solutions resulted in measurable levels of the compound in the breath, reaching a maximum 10 minutes after exposure (16). Experiments in hairless guinea-pigs have shown substantial dermal absorption from dilute aqueous solutions (21). In vitro dermal absorption of PCE in aqueous solution through freshly prepared and previously frozen human skin was measured and compared to absorption of other volatile organic compounds in aqueous solution (42). The dermal absorption of PCE from a soil matrix was compared in rats and humans using real-time mass spectrometric exhaled breath technology and physiologically based pharmacokinetic (PBPK) modelling (43).
Distribution
Repeated inhalation exposure to PCE results in accumulation of the compound in the body, especially in fatty tissue since it is lipid-soluble. PCE crosses the placenta and can be found in breast milk; the fetus and nursing neonates may therefore be at increased risk of adverse effects from maternal exposure (2,44,45). In rats, distribution to the brain, liver and kidneys has also been demonstrated (2,46). In a study in mice, transplacental transport of unchanged PCE has been observed (2). In rats and goats, it has been shown that excretion of unchanged PCE in milk occurs after intraruminal administration (23,24). Following inhalation exposure of lactating rats to a concentration of 4070 mg/m3 (600 ppm) for 2 hours, total body burden for the pups was up to about 14 mg/kg body weight (23). Since women have a higher proportion of body fat than men, it is assumed that women retain PCE longer (47).
Biotransformation
In experiments in humans and rats, it was observed that most of the absorbed amount of PCE is exhaled unchanged. The two principal pathways of PCE metabolism that occur in the liver and kidney of experimental animals are cytochrome P450-dependent oxidation and glutathione conjugation. The hepatotoxic, nephrotoxic and carcinogenic effects of PCE depend on its metabolism to reactive metabolites, which may covalently bind to cellular macromolecules.
Cytochrome P450 enzymes primarily catalyse PCE oxidation. Presumably, CYP2E1 plays an important role in this process in rodent liver and kidney as well as human liver. This results in the formation of a PCE-epoxide, which further reacts to trichloroacetyl chloride. Trichloroacetyl chloride can react with amino groups in macromolecules, resulting in hepatotoxicity. When it reacts with water it forms trichloroacetic acid.
Trichloroacetic acid is the principal metabolite recovered from urine in both humans and rodents following inhalation exposure to PCE. Another metabolite that can be formed by the cytochrome P450 pathway is dichloroacetate. Di- and trichloroacetate are associated with hepatic toxicity and carcinogenicity. Other metabolites of PCE by the P450 pathway that have been identified are shown schematically in Fig. 9.1(48,49).
There is a broad range of halogenated hydrocarbons and other small organic molecules that are oxidized by CYP2E1. Several drugs as well as physiological or pathological conditions may lead to the induction of CYP2E1. This implies that under certain conditions, prior or concurrent exposure to chemicals such as ethanol or acetaminophen may influence the response to PCE. Also, individual susceptibility due to genetic polymorphisms in CYP450-dependent metabolism can result in altered toxicity.
PCE is conjugated by glutathione to S-(1,2,2-trichlorovinyl)glutathione (TCVG). No significant effects were found of PCE or TCVG on cytotoxicity or mitochondrial function in isolated hepatocytes from rats or in isolated liver mitochondria from rats or mice. Therefore, it is unlikely that the liver is a major acute target for PCE or TCVG. Glutathione conjugation of PCE, followed by activation of trichlorovinylcysteine (TCVC), is responsible for nephrotoxicity and possibly nephrocarcinogenicity (49,50). Fig. 9.2 shows the metabolites of PCE by the glutathione conjugation pathway, so far as they have been identified to date.
Species-dependent differences in biotransformation
Mice metabolize PCE to trichloroacetic acid to a greater extent than rats. In rats, saturation of the oxidative metabolism at exposure concentrations exceeding 678 mg/m3 (100 ppm) prevents the occurrence of the high trichloroacetic acid concentrations that are observed in mice at these dose levels. The available evidence indicates that in humans too, saturation of the oxidative metabolism of PCE occurs at ≥ 678 mg/m3 (≥ 100 ppm) (51). In a comparative study, the urinary levels of N-acetyl-S-(1,2,2-trichlorovinyl)-L-cysteine, one of the final metabolites in the glutathione biotransformation pathway, were shown to be higher in rats than in mice after inhalation exposure to PCE. In vitro tests in hepatocytes and kidney fractions of rats, mice and humans suggest that the potential for this biotransformation in the kidneys is also lower in humans than in rats (2,6,52). Experimental data show that rates of TCVG formation in rats and mice are higher in males than in females, the formation rates in mice being higher than in rats (48,53).
The overall kinetics of PCE oxidative metabolism differs significantly between humans and rodents. The excretion of trichloroacetic acid after inhalation of PCE has been shown to be slower in humans than in rats. The elimination half-time of trichloroacetic acid in the urine was approximately 4.1-fold longer in humans than in rats exposed to 10 or 40 ppm PCE. Maximal trichloroacetic acid concentrations in blood were 3- to 10-fold lower (depending on dose) in humans than in rats (48,54). It is assumed that saturation of PCE metabolism occurs at lower doses in humans (55,56).
While the target organs for toxicity in general are similar for different species, this is not the case for carcinogenicity.
Sex-dependent differences in biotransformation
Regarding renal toxicity, sex differences have been found in experiments on rats and mice. PCE is demonstrated to cause a significant release of lactate dehydrogenase in isolated kidney cells from male but not from female rats. TCVG causes much more lactate dehydrogenase to be released from male than from female rat kidney cells. Examination of effects on mitochondrial respiration in suspensions of isolated mitochondria showed that PCE and TCVG are more toxic in renal mitochondria from male rats than in those from female rats. Respiratory function in (renal) mitochondria from mice was significantly inhibited by PCE and TCVG, but showed little sex dependence (49). Experimental data showed that TCVG formation in kidney and liver subcellular fractions from male rats and mice were invariably higher than corresponding values in female rats and mice (53).
Elimination
In humans and animals, the major part of the absorbed amount of PCE is exhaled unchanged. In humans, 80–100% of the uptake was exhaled as parent compound in the 7 days following a single 4-hour inhalation exposure to 488 or 976 mg/m3 (72 or 144 ppm) PCE. In rats, the proportion is somewhat lower (68%). Elimination of PCE from adipose tissue is relatively slow (calculated half-life 55 hours) owing to the high adipose/blood partition coefficient and the low rate of blood perfusion to this tissue (2). Excretion of metabolites in urine represents only a small proportion of the inhaled dose. Following single inhalation exposure in humans, only 2% of the uptake was found as the major urinary metabolite, tetrachloroacetic acid. This compound was excreted from the blood with a half-life of 75–80 hours. In another study, its half-life in urine was estimated at 6 days (16).
In a study in which humans were exposed by inhalation to 6.8 mg/m3 (1 ppm) PCE in the air for 6 hours, the average recovery by exhalation was calculated to be 82%. This implies that about 18% was metabolized. PCE was readily detected in alveolar air and venous blood; trichloroacetic acid was found in venous blood and urine. Recovery was primarily through exhaled air; only a small amount (less than 1% of intake) of trichloroacetic acid was excreted via the urine. Between about 63% and 93% of the intake was exhaled as parent compound 6 days after the exposure; 0–10% was estimated to be exhaled as PCE after the 6-day period (57). An inhalation study in humans and rats exposed for 6 hours at different concentrations of PCE showed excretion of trichloroacetic acid and the acetylated metabolite N-acetyl-TCVC in the urine of both species. Excretion of the N-acetyl-TCVC was significantly higher in male than in female rats (54).
In rat studies, high concentrations in the milk have been observed, peak concentrations being an order of magnitude higher than in maternal blood (58). Experimental data in lactating goats showed that, following intraruminal administration of an equal volume mixture of PCE, TCE and methyl chloroform, the milk secreted during the 24 hours following the administration contained 6.43 mg PCE (in addition to TCE and methyl chloroform). PCE demonstrated the greatest tissue partitioning compared to TCE and methyl chloroform, being persistently in the blood and secreted into the milk (59). Limited data in humans also show that PCE is excreted in the milk (60).
Biomarkers of exposure
Biological monitoring of exposure to PCE can be carried out by measuring levels of the parent com pound in blood, urine or exhaled air or of the metabolites in blood or urine. These methods have been applied for assessing both occupational and non-occupational exposure.
There has been discussion in the literature as to whether measurement of the parent compound in blood or in exhaled air is the preferred biological index to monitor PCE exposure (47,61–63). A study in humans showed that correlation coefficients (r) between environmental PCE and PCE-B (PCE measured in blood), PCE-Alv (PCE measured in alveolar air) and PCE-U (PCE measured in urine) were 0.94, 0.81 and 0.67, respectively. A high correlation was also found among biological indices: the r value was 0.96 between PCE-B and PCE-Alv, 0.95 between PCE-B and PCE-U, and 0.87 between PCE-Alv and PCE-U (61). The examined biological indices proved sensitive enough for biological monitoring of low exposure to PCE and can give substantially similar information in terms of exposure evaluation. PCE measurement in alveolar air offers some practical advantages over the other exposure indices.
Measuring of metabolites (trichloroacetic acid, trichlorethanol) in blood or urine may not necessarily represent exposure to PCE because some related chlorinated hydrocarbons (TCE, 1,1,1-trichloroethane) are converted to the same metabolites (2).
Physiologically based pharmacokinetic modelling
Several groups of researchers have developed PBPK models for PCE and several of the models have been evaluated. Table 9.1 provides an overview of these efforts (41,46,58,60,64–79).
Table 9.1
Physiologically based pharmacokinetic (PBPK) models for PCE metabolism.
Most PBPK models for PCE share the four-compartment structure (liver, fat, rapidly perfused tissues and slowly perfused tissues) and steady-state description of lung equilibration developed by Ramsey & Andersen (80) for styrene. Only one of the published models (71) provides a description of the kinetics of trichloroacetic acid, the major metabolite of PCE.
Some studies have focused on the evaluation of these models. In one, the key parameters and predictions for PBPK models for mice, rats and humans developed by different groups of researchers were compared. The amounts of metabolized PCE predicted showed considerable differences (about 12- to 14-fold for humans). Most of the differences in risk-related model predictions were due to the choice of the data sets used for calibrating metabolic parameters (Vmax, Km) (77,81). A statistical approach to some of the PBPK models for PCE in mice, rats and humans showed that the kinetic parameters defining the metabolic rate were the most important parameters for model sensitivity (82).
Health effects
Identification of studies
Epidemiological studies on health effects of PCE exposure were identified from electronic searches and hand searches of references in former reviews by WHO. Electronic searches were made in PubMed in July 2008, with an update in June 2009. We intended to identify all studies with original data on health effects of PCE and the main descriptors used were “tetrachloroethylene” and “perchloroethylene”. In addition, studies that examined the health effects of PCE were identified through hand searches of earlier reviews of the topic, citations within papers identified on health effects of PCE and searches on the web sites of international or national health assessment agencies, including WHO, the European Commission, Health Canada, IARC, ATSDR, USEPA, the United Kingdom Committee on Carcinogenicity of Chemicals in Food, Consumer Products and the Environment and the US National Institute for Occupational Safety and Health. Papers known to the expert group in 2009 concerning health effects of PCE were also used.
Of 148 papers identified, 59 were relevant to the health effects section of this chapter. These papers were included in the discussion presented below, addressing human exposure and epidemiological issues (37), children (0), animal studies (10), cell studies (4), reviews (4) and other studies (4). In addition, 11 reports of the above-mentioned agencies were used in the “Health effects” section.
Effects on experimental animals and in vitro test systems
Toxicological effects
PCE has a low acute inhalation toxicity (LC50 in rodents ≥ 16.6 mg/litre). Acute oral toxicity is also low (LD50 in rodents ≥ 3000 mg/kg body weight) (6).
The liver, kidneys, blood and CNS are the target organs for systemic effects. Hepatic effects occur at lower dose levels in mice than in rats. In a series of experi ments in mice, continuous (24 hours/day) inhalation exposure for 30 days at concentrations of 61, 251, 508 or 1017 mg/m3 produced a dose-related increase in liver weight at all dose levels. Morphological changes in the liver were observed at 251 mg/m3 and, as stated but not fully reported, at all other dose levels as well. Plasma butyrylcholinesterase activity showed a slight increase at ≥ 251 mg/m3. The reversibility of the effects was exami ned at 1071 mg/m3 only, showing that at 120 days after cessation of treatment, only a slight increase in liver weight remained (83).
Within the United States National Toxicology Program (NTP) subchronic inhalation studies were carried out in rats and mice. In mice, liver effects were observed at 1350 mg/m3 (≥ 200 ppm) or higher. Karyomegaly of the renal tubule epithelial cells was observed at ≥ 1350 mg/m3 (≥ 200 ppm). At 680 mg/m3 (100 ppm), no effects were seen but at this level no histopathological examination of the liver was carried out. In rats, mild congestion of the liver was seen at ≥ 1350 mg/m3 (≥ 200 ppm) (lower concentrations not tested), with congestion of the lungs, decreased survival and growth retardation at higher dose levels (84). In chronic studies, renal tubular cell karyomegaly was observed in female rats and mice at both 1356 and 2712 mg/m3 (200 and 400 ppm) in rats and 678 and 1356 mg/m3 (100 and 200 ppm) in mice.
In addition, in rats, renal tubular hyperplasia (males), thrombosis and squamous metaplasia in the nasal cavity (males and females) and hyperlasia in the adrenal medullae (males) or cortices (females) were found. In mice only, liver cell degeneration and necrosis were seen at both concentrations (84). A study exposing mice to 2040 mg/m3 (300 ppm) perchloroethylene gas for 6 hours daily for 5 days revealed regeneration of olfactory mucosa. Two weeks and three months after exposure, the nasal mucosa was examined histopathologically. After two weeks, ciliated epithelium started to replace the olfactory epithelium. Damage was more persistent in the nasal mucosa of the olfactory region than in the respiratory region (85).
CNS effects have been observed at high dose levels. In the short-term NTP experiment in rats and mice, neurological symptoms were observed only at the highest concentration of 11 860 mg/m3 (1750 ppm) (84). Biochemical changes in brain tissues have been observed in rats and gerbils at concentrations of ≥ 407 mg/m3 (≥ 60 ppm). However, the toxicological significance of these changes is unclear (2). In a recent short-term study in rats, decreased weight of brain regions and reductions in neuronal marker proteins were seen at 4080 mg/m3 (600 ppm) but not at 2040 mg/m3 (300 ppm) (86). Haematotoxicity was observed in an inhalation study in mice at test concentrations of 915 and 1830 mg/m3 (no other concentrations tested) in which the animals were exposed for 6 hours/day, 5 days/week for 7.5 or 11.5 weeks (87). Rats that were acutely exposed to a high dose (500 mg/kg oral) of PCE and others subchronically to lower doses (5 and 50 mg/kg oral), showed significant effects on nociception and locomotor activity (88). An inhalation study assessed the effects of PCE on sustained attention in rats performing a visual signal detection task. It showed that inhaled PCE acutely impaired sustained attention in rats, and its potency weakened on repetition of the exposure. Accuracy was significantly reduced at 3390 mg/m3 (500 ppm), significantly elevated response time was seen at 6780 mg/m3 (1000 ppm) and significantly reduced trial completions were found at 10 170 mg/m3 (1500 ppm) (89).
Limited teratogenicity studies in rats, mice and rabbits suggest that PCE produces fetotoxicity and embryotoxicity at high dose levels (≥ 2034 mg/m3). In a behavioural teratogenicity study in rats, neuromuscular ability in pups was decreased at 6100 mg/m3 (900 ppm) but not at 2034 mg/m3 (300 ppm) (2). In a study on developmental toxicity, embryos from rats were explanted on gestational day 120 and cultured for 46 hours in the presence of PCE. Exposure to PCE ranging from 3.5 to 15 mM caused concentration-related effects on growth and development. The presence of hepatic microsomal fractions in the culture medium partially prevented the effects of PCE on the measured parameters of embryo growth and development (90). A study showed altered behaviour in adult mice orally exposed to PCE as neonates (5 and 320 mg/kg PCE/day between days 10 and 16 postnatally), indicating neonatal susceptibility of brain maturation in achieving long-lasting changes in adult behaviour (91). A developmental study in rats following inhalation exposure to PCE showed maternal responses that were of limited toxicological significance. Developmental effects at 600 ppm consisted of reduced gravid uterus, placental and fetal weights and decreased ossification of thoracic vertebral centra. Developmental effects at 1695 mg/m3 (250 ppm) were of minimal toxicological significance, being limited to minor decreases in fetal and placental weights. There were no developmental effects at 441 mg/m3 (65 ppm) (92).
PCE at 68–2043 mg/m3 (10–300 ppm) was found to increase, in a concentration-dependent manner, ACh- and high K+-induced muscle contraction in tracheal tissue from swine. Swine tissue was used because there are many similarities between swine and human airways. More research is necessary to be able to conclude that these responses play a role in airway impairment and hyperresponsiveness after PCE exposure (93). A study using renal cell suspensions of rats and mice showed that PCE caused acute renal cellular toxicity in rats and mitochondrial toxicity in rats and mice (49).
Carcinogenic effects
Data on the carcinogenicity of PCE have been evaluated in various health assessment documents (2,6,51). PCE-induced nephrotoxicity and nephrocarcinogenicity have been associated with metabolism by the glutathione conjugation pathway to form TCVG (48,53).
Oral and inhalation studies with NTP have been performed in mice and rats. In a gavage study in B6C3F1 mice (time-weighted average (TWA) daily dose levels of 536 and 1072 mg/kg body weight in males and 386 and 772 mg/kg in females), increased incidences of hepatocellular carcinomas were found in males and females (4/37, 32/49 and 27/48 in males and 2/40, 19/48 and 19/48 in females). In Osborne Mendel rats, no increase in tumour incidence was seen. This study has various limitations: numerous dose adjustments during the study, early mortality related to compound-induced toxic nephropathy, and pneumonia due to intercurrent infectious disease in both rats and mice (2,94). In the inhalation study in B6C3F1 mice (test concentrations 100 and 200 ppm, 6 hours/day, 5 days/week for 103 weeks, corresponding to 680 and 1360 mg/m3), incidences of hepatocellular carcinomas were increased (7/49, 25/49 and 26/50 males and 1/48, 13/50 and 36/50 females). In F344/N rats (test concentrations 200 and 400 ppm, 6 hours/day, 5 days/week for 103 weeks, corresponding to 1360 and 2720 mg/m3), PCE caused dose-related increases in the incidence of stage 3 mononuclear cell leukaemia in animals of both sexes: in males, 20/50 in controls, 24/50 at the low dose and 27/50 at the high dose; in females, 10/50, 18/50 and 21/50, respectively. The historical control for mononuclear cell leukaemia in rats in the same laboratory was 47% in males and 29% in females. Uncommonly occurring renal tubular cell adenomas or adenocarcinomas were found in male rats (adenomas,1/49 in controls, 3/49 at the low dose and 2/50 at the high dose; adenocarcinomas, 0/49, 0/49 and 2/50, respectively).
Although induction of peroxisome proliferation as a mechanism underlying the hepatocarcinogenic effect of PCE in mice appears attractive, a poor quantitative correlation was seen between peroxisome proliferation and tumour formation in the liver following administration by inhalation.
The induction of kidney tumours observed in male rats provides only weak evidence for (human) carcinogenicity. The observed increase in incidence is not statistically significant. In addition, two different mechanisms of action have been proposed for the induction of these tumours: alpha 2μ-globulin nephropathy, an effect specific for male rats, and formation of genotoxic metabolites in the kidneys as the final step of the glutathione biotransformation pathway. Given the low incidences observed, combined with the data on the mechanism of induction, it can be concluded that the result in male rats is equivocal evidence only for a risk of renal cancer in humans.
A review of six carcinogenicity studies of PCE in rats concluded that evidence of increases in mononuclear cell leukaemia has been seen in two studies in the F344 rat but not in other strains, and that as a rat-strain-specific effect it is not considered to be relevant to the evaluation of human cancer risk (95).
IARC (96) has concluded that the results of the available animal bioassays provide sufficient evidence for carcinogenicity in animals.
Mutagenicity
Comprehensive reviews are available of the data obtained from the many studies carried out with PCE (2,51,96). In vitro studies include tests for gene mutations in prokaryotes and eukaryotes and tests for chromosome aberrations in mammalian cell lines. In vivo studies include tests for chromosome aberrations in bone marrow in rats and mice and a dominant-lethal assay in rats. In addition, several studies have been carried out on DNA damage in vitro and in vivo. The body of results shows absence of mutagenicity of PCE in virtually all of the systems tested. The few weakly positive results may be due to the presence of mutagenic stabilizers in the test samples (2,51). Binding of PCE in vivo to unpurified DNA from several organs was noted in one study, although no covalent binding to purified hepatic DNA could be demonstrated in another. In the other available in vivo studies, it is not known whether the test compound (or its metabolites) reached the target tissues. This observation reduces the value of the negative results observed. Nevertheless, an in vivo study, in which male mice were administered PCE intraperitoneally, assessed the induction of micronuclei in hepatocytes and reticulocytes and showed that PCE might have a clastogenic effect in hepatocytes (97). For the metabolites of PCE that are formed by conjugation with glutathione (a minor biotransformation route demonstrated in rodents), positive results were obtained in in vitro studies in S. typhimurium TA 100 (reverse mutation), with metabolic activation with kidney microsomes, in the presence of glutathione and glutathione transferase (96).
Interactions with other chemicals
Results of studies on the influence of ethanol on the metabolism and toxicity of PCE in liver did not show synergism (2,98). Increased urinary excretion of metabolites of PCE and enhanced hepatotoxicity have been observed in rats following pretreatment with polychlorinated biphenyls (2). Simulation of interaction thresholds for human exposure to mixtures of TCE, PCE and 1,1,1-trichloroethane was done with PBPK models. Model simulations indicated that during combined exposures, certain toxicological effects may be expected to occur at lower exposure levels compared with PCE exposure alone (73,99).
Effects on humans
Exposure to PCE can affect the CNS, eyes, kidney, liver, lungs, mucous membranes and skin. CNS effects have been most frequently noted (44).
The odour threshold in air for detection of PCE is 8 mg/m3. The odour threshold in air for recognition of PCE is 3–4 times higher (16).
Acute poisoning incidents
Acute exposure to PCE at air levels of 100–200 ppm can cause irritation of the skin, eyes and upper respiratory tract. Non-cardiogenic pulmonary oedema, nausea, vomiting and diarrhoea can occur. CNS effects have also been observed with acute inhalation exposure to PCE at 50–300 ppm. At these levels, neuromotor effects may be seen and results of certain coordination and behavioural tests may be abnormal. At higher air concentrations, loss of consciousness can occur (44).
Several acute PCE intoxications have been reported. In one case, up to 16 mg was ingested by a 6-year-old boy weighing 22 kg. He was admitted to hospital one hour after ingestion, where his condition deteriorated from somnolence to coma. The initial blood level was 21.5 μg/ml. He recovered completely without liver, renal or CNS injury and was discharged on day 9 (100). In another report, a 32-year-old man became semi-comatose and experienced oliguric acute renal failure after accidental ingestion of 75 g PCE. A renal biopsy performed on the 19th day after ingestion showed severe acute tubular necrosis. He regained normal renal function after five haemodialyses and conservative treatment. The amount of PCE in the blood and urine were not measured (101).
Three fatalities due to inhalation of PCE have been reported in the literature. A concentration of 4.5 mg/l was measured in the blood of a man aged 53 years weighing 70 kg, who died while he was cleaning and recycling PCE by distillation (102). A two-year-old boy died due to PCE that had been retained in the curtains of his bedroom. A concentration of 66 mg/l blood was measured (103,104). Finally, a 33-year-old man clearing a line in a dry cleaning establishment died with a blood concentration of 44 mg/l (105). A fatality due to poisoning by both PCE and TCE has been described, a 45-year-old woman being discovered unconscious and in cardiac arrest in a laundry area. Examination revealed that she was deeply comatose and had acute respiratory distress syndrome and severe metabolic acidosis. Cardiovascular instability and acute renal failure occurred and eventually the patient died; the results of the autopsy are described in the paper. The blood levels of PCE 2, 4, 5, 6 and 7 days after hospital admission were respectively 1319, 758, 787, 436 and 656 μg/l (106).
Effects on the CNS
Depending on the concentration, acute exposure can result in loss of coordination, reversible mood and behavioural changes, or potentially anaesthetic effects. People chronically exposed to PCE may experience ataxia, disorientation, irritability, peripheral neuropathy, short-term memory deficits and sleep disturbance. Reversibility depends on the degree of severity of the exposure and associated effects (44).
PCE has been associated with neurobehavioural dysfunction, including reduced attention in humans. In a series of controlled short-term studies in limited numbers of human volunteers, neurological symptoms (including dizziness, drowsiness and decreased functioning in motor coordination tests) and visual system dysfunction were observed at ≥ 678 mg/m3 (≥ 100 ppm) and 339 mg/m3 (50 ppm), respectively (2,16,107,108). Limited information on neurological effects following long-term exposure was obtained in occupational studies in dry cleaning workers. In a cross-sectional study with two groups of exposed workers (exposure concentrations 83 ± 53 mg/m3 and 364 ± 114 mg/m3, duration of exposure 127 or 141 days), small effects on scores in psychological tests were found. However, the response did not correlate with the exposure level in this study (2,6,109).
In another study in female dry cleaning workers with exposures of 6.8–408 mg/m3 (4-hour averages, median 102 mg/m3) and PCE concentrations in the blood of 12–864 mg/l (median 145 mg/l), performance test scores in a test battery for neuromotor functions were decreased. Neither duration of exposure nor blood concentration of PCE was significantly correlated with performance (110). In cross-sectional studies in dry cleaning workers, effects on blue–yellow colour vision were found at mean PCE concentrations of 42–102 mg/m3(111,112). In Italy, PCE-induced impairment of colour vision was studied in 33 dry cleaning workers. Exposure was evaluated with passive samplers and colour vision was assessed. Two years later, the workers were re-examined. The exposure to PCE had increased in subgroup A (median 1.7 ppm vs 4.3 ppm). In subgroup B, exposure was reduced (median 2.9 ppm vs 0.7 ppm). Colour vision worsened in subgroup A but no vision changes were noticed in subgroup B, indicating that an increase in exposure during a 2-year period can cause colour vision to deteriorate (113).
A case report described a 57-year-old woman exposed to supposedly high concentrations of PCE while working at a dry cleaning shop, who suffered from blindness for 9 days in the left eye and 11 days in the right eye with optic neuritis. Blood concentrations of PCE 48 and 80 hours after onset of the optic neuritis were respectively 1.08 mg/g and 0.65 mg/g (114).
In a prospective population-based cohort study on 88 829 children, offspring from dry cleaners (144 children) were followed from birth to age 21–33 years. Preliminary findings suggested an increased incidence of schizophrenia (4 cases) in these children (115). However, it should be mentioned that there was no exposure characterization, there were few cases and few details were provided. These findings need to be corroborated in follow-up studies.
Research from 2002 suggests that chronic, environmental exposure to airborne PCE adversely affects neurobehavioural function in healthy individuals. A significantly lower visual contrast sensitivity was reported in apartment residents exposed to PCE (daytime mean 620 μg/m3, nighttime mean < 100 μg/m3) compared to unexposed controls. The reliability of these data was later discussed in the literature. Methodological limitations preclude a definitive attribution of causation, and further research is necessary to draw reliable conclusions (99,116).
Effects on the liver
Case reports of human exposure to PCE show that it can cause hepatotoxic effects in humans, which include abnormal liver function tests (48), cirrhosis, hepatitis, hepatomegaly and liver cell necrosis (44). A dose–response relationship in humans for the effects on the liver is not completely known. In case studies of high accidental exposures, effects on the liver have been reported. In limited studies in dry cleaning workers exposed to a TWA concentration of 143 mg/m3 (21 ppm) over a 6-year period, serum enzymes indicative of liver function were not affected (62,112). A study in dry cleaners frequently exposed to PCE concentrations of 16 ppm (8-hour TWA) revealed mild to moderate hepatic parenchymal changes as determined by hepatic ultrasonography (117).
Renal effects
Nephrotoxic effects have been described in humans (48,118,119). Symptoms of renal dysfunction, including proteinuria and haematuria, have been associated with accidental exposure to anaesthetic concentrations of PCE vapour (2). In several cross-sectional studies in dry cleaning workers, the effect of PCE on renal function was examined. Female workers exposed for an average of 14 years to an estimated TWA concentration of 68 mg/m3 (10 ppm) had increased urinary levels of lysozyme and ß-glucuronidase suggestive of mild renal effects (120). No effects were observed in workers estimated to have been exposed to a TWA concentration of 142 mg/m3 (21 ppm) for 6 years (62). In a cross-sectional study, about 20 markers of early nephrotoxic effects were measured in workers in dry cleaning facilities (n = 50). Exposure was determined by analysing air samples collected during 4-hour periods randomly selected over the working week. The median exposure concentration was 102 mg/m3 (range, trace–580 mg/m3). Compared to the control population, the exposed group had significantly higher frequencies of abnormal values for a number of the markers in urine, including albumin, transferrin, tissue-nonspecific alkaline phosphatase and brush-border antigens. The significance of the findings cannot be easily assessed but may represent an early stage of clinically silent but potentially progressive renal disease (118). At an average airborne exposure of 8.4 mg/m3 (range 2.2–44.6), a PCE-related effect on the tubular reabsorption of retinol-binding protein in urine was observed in exposed workers from a dry cleaning shop (119).
Since there are sex- and species-dependent differences in bioactivation and detoxification pathways, more knowledge about the metabolism of PCE in several target organs and in different species is needed to improve human risk assessment (50).
Reproductive and developmental effects
A few epidemiological studies have reported reproductive or developmental abnormalities due to exposure to PCE in drinking-water. The 1998 Camp LeJeune study reported a weak association between PCE exposure due to contaminated drinking-water and birth weight outcomes for small-for-gestational age births. Stronger associations were observed between PCE exposure and birth weight for infants of mothers who were 35 years of age or older and for infants of mothers with a history of fetal death (121). Another study found oral cleft defects associated with PCE-contaminated drinking-water. The authors indicate that this study cannot resolve whether the drinking-water contaminants caused the adverse birth outcomes; these findings should therefore be followed up utilizing available drinking-water contamination databases (122).
In a comment on this paper, it is suggested that the oral cleft defects found are biologically plausible (123). According to these authors, a study of mothers with pregnancies affected by neural tube defects in Dublin (124) suggests a mechanism for the induction of neural tube defects related to a metabolic slowdown of the methionine synthase reaction. Recently, two cohort studies examined the effects of prenatal exposure to PCE-contaminated drinking-water on adverse birth outcome and risk of behavioural disorders. It was concluded that prenatal and early postnatal PCE exposure is not associated with disorders of attention, learning and behaviour (125). Further, it was suggested that prenatal PCE exposure does not have an adverse effect on birth weight or gestational duration (126).
The results of several studies have indicated that occupationally exposed women might suffer higher rates of spontaneous abortion (127–129). Exposure was classified by reported work history. A significant effect was found when the work tasks included dry cleaning for at least one hour daily or the women reported handling of PCE at least once a week (128,129). A significantly higher risk was found in operators compared to non-operators (127), but other studies have not found this association (130,131). Furthermore, a case-referent study performed within two cohorts did not give any substantial support to the hypothesis that exposure to PCE at work during pregnancy enhances the risk of adverse pregnancy outcome (132). A retrospective time-to-pregnancy study among Finnish women indicated a reduced ability to reproduce among 20 women exposed to PCE (concentration not clear) for 1–4 days a week or daily by inhalation. It should be noted that the studies have a number of limitations, primarily that exposure concentrations are not available (133).
The Camp Lejeune study of ATSDR (134) was not included in this guideline, since an error was made in the exposure classification; these data will be re-analysed by ATSDR at a later stage.
Mutagenic and carcinogenic effects
IARC concluded in 1995 that there is evidence for consistently positive associations between exposure to PCE and the risks for oesophageal and cervical cancer and non-Hodgkin's lymphoma. These associations appear unlikely to be due to chance, although confounding factors cannot be excluded and the total numbers in the cohort studies combined are relatively small. IARC therefore concluded that there is limited evidence for the carcinogenicity of PCE in humans (96).
PCE is reasonably anticipated to be a human carcinogen on the basis of limited evidence from studies in humans and sufficient evidence of carcinogenicity from studies in experimental animals. PCE has been studied by observing laundry and dry cleaning workers, who may also have been exposed to other solvents, especially TCE but also petroleum solvents. In several cohort and proportionate mortality studies, excesses have been reported of lymphosarcomas, leukaemias and cancers of the skin, colon, lung and urogenital tract. Some excess of lymphomas and of cancers of the larynx and urinary bladder was seen in a large cohort of dry cleaners. A familial cluster of chronic lymphocytic leukaemia has also been related to dry cleaning. Although these studies suggest a possible association between long-term occupational exposure to PCE and increased lymphatic malignancies and urogenital cancers, the evidence must be regarded as inconclusive because workers were exposed to petroleum solvents and other dry cleaning agents as well as PCE (135).
The results of a study in differently exposed dry cleaners and launderers indicated a reduction in oxidative DNA damage in exposed dry cleaners compared to launderers. The mean value for PCE in the blood of these dry cleaners was 0.078 mg/l, and the TWA was < 5 ppm in air for 17 out of 18 dry cleaners. However, PCE could not clearly be identified as the source of the effect (136). In two studies for genetic effects (sister chromatid exchanges and/or chromosome aberrations) in lymphocytes of occupationally exposed workers, no clear-cut effects were found (6). Another study showed modulation of the expression of some genes related to cancer induction in human cord blood cells (137).
A population-based case-control study was undertaken to investigate the association between breast cancer and PCE exposure from public drinking-water (drinking-water consumption as well as bathing habits were taken into account). The same author performed a more extensive case-control study a few years later. Both studies suggest that women with the highest PCE exposure levels have a slightly to moderately increased risk of breast cancer. Analyses of the same data using a newly constructed personally delivered dose model to make a more accurate estimation of the exposure did not significantly change the subjects' exposure classification (138–140). An association between PCE exposure from public drinking-water and lung cancer and possibly colorectal cancer was suggested, based on another population-based case-control study (141).
A paper from 2003 assessed (based on specified methodological and scientific quality criteria) 44 epidemiological papers that provided reasonable data on up to 17 cancer sites. The authors concluded that no evidence for an association between breast, prostate, skin or brain cancer and exposure to PCE was demonstrated. A relationship between PCE and cancer of the oral cavity, liver, pancreas, cervix and lung was considered unlikely by the authors. Scientific evidence was considered inadequate for laryngeal, kidney, oesophageal and bladder cancers (142).
In 2006, the epidemiological literature since 1990 on occupational chlorinated solvent exposure was reviewed. The paper reviewed 28 studies of PCE, all but 5 of which focused on workers in the dry cleaning industry with few or no other chemical exposures. Within the study populations with multiple exposures, three studies found some increased risk of biliary and liver or brain cancer; in the other studies, no increased risk for renal cell carcinoma or for any cancer was found. Census records for those working as a “dry cleaner or laundry worker” or from a database of those being monitored for PCE exposure were linked to cancer registries (three studies) and statistically significant increased risks for liver and pancreatic cancer, Hodgkin's lymphoma and leukaemia were found. In general, findings in occupational cohort studies have not been consistent, even within the same industries (143).
Health risk evaluation
Critical health outcomes
The main health effects of concern are local irritation (eyes, mucous membranes, respiratory tract and skin), effects on the CNS and cancer. Effects on the liver and kidneys have also been reported.
CNS effects and local irritation
The evidence is sufficient to conclude that PCE can cause irritation of mucous membranes and the respiratory tract. These effects generally occur at high concentrations. Acute exposure to PCE at air levels of 680–1360 mg/m3 (100–200 ppm) can cause irritation of the skin, eyes and upper respiratory tract in humans. Moreover non-cardiogenic pulmonary oedema, nausea, vomiting and diarrhoea can occur. CNS effects have been observed at high dose levels in rats and mice. In humans, CNS effects have also been observed with acute inhalation exposures of 340–2040 mg/m3 (50–300 ppm) of PCE. The evidence is sufficient to conclude that there is an association between PCE and CNS effects at sufficiently high concentrations. Since high concentrations typically do not occur in indoor environments for a long period of time, these end-points are not taken into account when setting the guideline value.
Liver and kidney effects
The evidence of an association between PCE exposure and effects on the liver is suggestive. Hepatic effects have been observed in rats and mice in subchronic studies. Case reports of accidental exposures of humans to PCE confirm that hepatotoxic effects can occur in humans, including abnormal liver function tests, cirrhosis, hepatitis, hepatomegaly and liver cell necrosis.
There is suggestive evidence of an association between PCE exposure and effects on the kidneys. In chronic studies, renal effects were observed at 1360 and 2710 mg/m3 (200 and 400 ppm) in rats and at 680 and 1360 mg/m3 (100 and 200 ppm) in mice. Reversible kidney damage in humans (e.g. proteinuria and haematuria) has been associated with accidental exposure to acutely toxic concentrations of PCE vapour. A long-term exposure study showed that renal effects may develop at lower concentrations, with the reported onset of renal damage occurring following exposure to a median concentration of 102 mg/m3 (range, trace–576 mg/m3).
Reproductive and developmental effects
A few epidemiological studies have reported developmental abnormalities due to PCE exposure in drinking-water (reduced birth weight and oral cleft defects). Other studies concluded that prenatal PCE exposure does not have an adverse effect on birth weight or gestational duration. Some studies have consistently indicated that occupationally exposed women might suffer higher rates of spontaneous abortion, while others found no association. All the above-mentioned studies have limitations, primarily that the exposure concentrations are not available. Therefore the evidence stays suggestive.
Cancer risk
IARC concluded that the results of the available animal bioassays provide sufficient evidence for carcinogenicity to animals. In these studies, an increased incidence of adenomas and carcinomas was observed in the livers of exposed mice. There is suggestive evidence from mechanistic studies that humans are likely to be less sensitive to the development of these tumours following PCE exposure. A low incidence of kidney tumours among male rats has been reported.
It can be concluded from this small and statistically non-significant increase, together with the data relating to the possible mechanism of induction, that the result in male rats is equivocal evidence only for a risk of renal cancer in humans.
The significance for humans of the increased incidence of mononuclear cell leukaemia, as observed in two studies in F344 rats, is unclear. This is due to the lack of understanding of the mechanism underlying the formation of this cancer type (which has a high background incidence) and the suggestion that it might be a rat-strain-specific effect.
IARC concluded in 1995 that there is evidence for consistently positive associations between exposure to PCE and the risks for oesophageal and cervical cancer and non-Hodgkin's lymphoma.
These associations appear unlikely to be due to chance, although confounding factors cannot be excluded and the total numbers in the cohort studies combined are relatively small.
A paper from 2003 assessed (based on specified methodological and scientific quality criteria) 44 epidemiological papers that provided reasonable data on up to 17 cancer sites. The authors concluded that no evidence for an association between breast, prostate, skin or brain cancer and exposure to PCE was demonstrated.
A relationship between PCE and cancer of the following sites was considered unlikely by the authors: oral cavity, liver, pancreas, cervix and lung. Scientific evidence was considered inadequate for laryngeal, kidney, oesophageal and bladder cancers. Since then, new studies have been published, but the evidence concerning the carcinogenicity of PCE is still not conclusive. The evidence for an association therefore remains suggestive.
From the weight of the evidence from mutagenicity studies, there are no indications that PCE is genotoxic. Although several in vitro studies indicate that conjugation of PCE with reduced glutathione (a minor biotransformation route demonstrated to occur in rodents) produces renal metabolites that are mutagenic in S. typhimurium TA 100, the significance of the latter results for humans is doubtful. In two studies investigating genetic effects (sister chromatid exchanges and/or chromosome aberrations) in lymphocytes of occupationally exposed workers, no clear-cut effects were found.
In conclusion, carcinogenicity is not used as an end-point, since there are no indications that PCE is genotoxic and there is some uncertainty about the epidemiological evidence as well as the relevance of the animal carcinogenicity data to humans. However, because of the remaining uncertainty about the carcinogenicity of PCE, it should be kept under review.
Health relevance of current indoor exposures
Inhalation of PCE is the major route of exposure in the general population. Ambient air concentrations of PCE are generally < 5 μg/m3 in urban areas and typically < 1 μg/m3 in rural areas. Indoor concentrations are generally well below 20 μg/m3, with median concentrations ranging from 0.16 to 8.7 μg/m3 in the described studies. These concentrations are an order of magnitude below concentrations that can be relevant for health.
Indoor PCE air levels may be up to 5.0 mg/m3 (4–24-hour average) in buildings with dry cleaning operations where PCE is used. There is sufficient evidence to conclude that concentrations in premises next to dry cleaning operations can exceed safe concentrations. This should be the focus of concern.
Conclusions of other reviews
IARC concluded that there is evidence for consistently positive associations between exposure to PCE and the risks for oesophageal and cervical cancer and non-Hodgkin's lymphoma. PCE is classified by IARC as a Group 2A carcinogen (probably carcinogenic to humans) (96).
The EU classified the substance as carcinogenic category 3 (substances that cause concern for humans owing to possible carcinogenic effects but in respect of which the available information is not adequate for making a satisfactory assessment) and risk phrase 40 (limited evidence of a carcinogenic effect) (144).
USEPA does not currently have a classification for the carcinogenicity of PCE but has calculated a provisional inhalation unit risk estimate of 5.8 × 10−7 (μg/m3)−1. A provisional value is one that has not received Agency-wide review (145).
The United Kingdom Committees on Toxicity, Mutagenicity and Carcinogenicity of Chemicals in Food, Consumer Products and the Environment concluded that there was “no satisfactory epidemiological evidence to associate PCE exposure to cancer in the available cohort studies” (146).
The US National Institute for Occupational Safety and Health considers PCE to be a potential occupational carcinogen (147).
ATSDR has derived both an acute inhalation minimal risk level (MRL) of 1.38 mg/m3 (0.1 ppm) and a chronic inhalation MRL of 0.28 mg/m3 1 (0.04 ppm) (2).
Guidelines
Carcinogenicity is not selected as the end-point for setting the guideline value for three reasons: the epidemiological evidence is equivocal, the animal tumours detected are not considered relevant to humans, and there are no indications that PCE is genotoxic. The derivation of a guideline value is at present based on two non-neoplastic effects as the critical end-point: impaired neurobehavioural performance and early renal changes.
On the basis of a long-term LOAEL for kidney effects of 102 mg/m3 in dry cleaning workers, a guideline value of 0.25 mg/m3 has been calculated. In deriving this guideline value, the LOAEL is converted to continuous exposure (dividing by a factor of 4.2 (168/40)) and divided by an uncertainty factor of 100 (10 for use of a LOAEL and 10 for intra-species variation). Recognizing that some uncertainty in the LOAEL exists because the effects observed at this level are not clear-cut and because of fluctuations in exposure levels, an alternative calculation was made based on the LOAEL in mice of 680 mg/m3 and using an appropriate uncertainty factor of 1000. This calculation yields a guideline value of 0.68 mg/m3.
A chronic inhalation MRL of 0.28 mg/m3 (0.04 ppm) has been derived by ATSDR based on the LOAEL of 15 ppm identified in the Ferroni study (110). The MRL was calculated from this concentration by expanding to continuous exposure (8/24 hours, 5/7 days) and dividing by an uncertainty factor of 100 (10 for use of a LOAEL and 10 for human variability). This reference found significantly prolonged reaction times in workers occupationally exposed to an average of 15 ppm for about 10 years (2,110).
The value and appropriateness of establishing a short-term guideline value is questionable because acute effects occur only at very high concentrations of 50 ppm (340 mg/m3) and higher, compared to generally observed levels in close proximity to dry cleaning facilities. Establishing a long-term value is more protective of human health.
On the basis of the overall health risk evaluation, the recommended guideline for year-long exposure is 0.25 mg/m3. This is the same as the previous WHO guideline (148).
The guidelines section was formulated and agreed by the working group meeting in November 2009.
Summary of main evidence and decision-making in guideline formulation
Critical outcome for guideline definition
Effects in the kidney indicative of early renal disease and impaired neurobehavioural performance. Carcinogenicity is not used as an end-point as there are no indications that PCE is genotoxic and there is uncertainty about the epidemiological evidence and the relevance to humans of the animal carcinogenicity data.
Source of exposure–effect evidence
- –
Based on a health surveillance study of dry cleaning workers exposed to PCE, the long-term LOAEL for kidney effects was considered to be 102 mg/m3. This was adjusted for continuous exposure by dividing by a factor of 168/40. Further, factors of 10 for use of a LOAEL and 10 for intra-species variation were incorporated, leading to a guideline value of 0.25 mg/m3(62).
- –
Based on a study of 30 female dry cleaning workers exposed to PCE for an average of 10 years (110), a LOAEL of 103 mg/m3 (15 ppm) has been derived and a chronic-duration inhalation MRL of 0.28 mg/m3 (0.04 ppm) calculated.
Supporting evidence
- –
Several epidemiological studies on renal changes and exposure to PCE, including studies in dry cleaning facilities. The significance of these findings cannot be easily assessed, but may represent a relationship between long-term exposure and effects on the kidney in humans (48, 118,119, 120).
- –
Associations between PCE exposure and neurobehavioural symptoms were suggested in two studies (108,109).
Results of other reviews
Guideline
0.25 mg/m3 – annual average.
Comments
No change in the guideline as compared to Air quality guidelines for Europe (148).
References
- 1.
- Hazardous Substances Data Bank (HSDB) [online database]. Bethesda, MD: National Library of Medicine; 2010. [19 May 2010]. http://toxnet
.nlm.nih .gov/cgi-bin/sis/htmlgen?HSDB. - 2.
- Agency for Toxic Substances and Disease Registry (ATSDR). Toxicological profile for tetrachloroethylene. Atlanta, GA: US Department of Health and Human Services; 1997.
- 3.
- Verschueren K. Handbook of environmental data on organic chemicals. 4th ed. New York, NY: John Wiley & Sons; 2001.
- 4.
- KowWin Estimation Software, ver. 1.66. North Syracuse, NY: Syracuse Research Corporation; 2002.
- 5.
- AopWin Estimation Software, ver. 1.90. North Syracuse, NY: Syracuse Research Corporation; 2002.
- 6.
- Tetrachloroethylene. Priority substances assessment report for the Canadian Environmental Protection Act. Ottawa: Environment Canada and Health Canada; 1993.
- 7.
- Howard PH, et al. Handbook of environmental degradation rates. Boca Raton, FL: Lewis Publishers; 1991.
- 8.
- Dry cleaning, some chlorinated solvents and other industrial chemicals Summary of data reported and evaluation. Lyon: International Agency for Research on Cancer; 1995. (IARC Monographs on the Evaluation of Carcinogenic Risks to Humans, Vol. 63)
- 9.
- Development of initial risk assessment method for chemical substances and preparation of the initial risk assessment. Annual report 2002. Tokyo: National Institute of Technology and Evaluation; 2003.
- 10.
- Von Grote J, et al. Reduction of occupational exposure to perchloroethylene and trichloroethylene in metal degreasing over the last 30 years: influences of technology innovation and legislation. Journal of Exposure Analysis and Environmental Epidemiology. 2003;13:325–340. [PubMed: 12973361]
- 11.
- Lewis RG, Gordon SM. Sampling for organic chemicals in air. In: Keith LH, editor. Principles of environmental sampling. 2nd ed. Washington, DC: American Chemical Society; 1996. pp. 401–470.
- 12.
- Mudder TI, Musterman JL. Development of empirical structure biodegradability relationships and biodegradability testing protocol for volatile and slightly soluble priority pollutants. Abstracts of Papers of the American Chemical Society. 1982;184:18.
- 13.
- Bouwer EJ, Rittmann BE, Mccarty PL. Anaerobic degradation of halogenated 1-carbon and 2-carbon organic-compounds. Environmental Science & Technology. 1981;15:596–599. [PubMed: 22283955]
- 14.
- Chemicals in the environment. Report on environmental survey and wildlife monitoring of chemicals. Tokyo: Ministry of the Environment; 2001. [4 July 2010]. http://www
.env.go.jp /chemi/kurohon/en/http2001e/index.html. - 15.
- Aggazzotti G, et al. Indoor exposure to perchloroethylene (PCE) in individuals living with dry-cleaning workers. Science of the Total Environment. 1994;156:133–137. [PubMed: 7992032]
- 16.
- Tetrachloroethylene. Geneva: World Health Organization; 1984.
- 17.
- Vartiainen T, et al. Population exposure to trichloroethene and tetrachloroethene and cancer risk – 2 cases of drinking-water pollution. Chemosphere. 1993;27:1171–1181.
- 18.
- Database of Water Quality of Aqueduct [online database]. Tokyo: Japan Water Works Association; 2008. [4 July 2010]. http://www
.jwwa.or.jp/mizu/list.html. - 19.
- Adgate JL, et al. Outdoor, indoor, and personal exposure to VOCs in children. Environmental Health Perspectives. 2004;112:1386–1392. [PMC free article: PMC1247565] [PubMed: 15471730]
- 20.
- Adgate JL, et al. Personal, indoor, and outdoor VOC exposures in a probability sample of children. Journal of Exposure Analysis and Environmental Epidemiology. 2004;14:S4–S13. [PubMed: 15118740]
- 21.
- Clayton CA, et al. National Human Exposure Assessment Survey (NHEXAS): distributions and associations of lead, arsenic and volatile organic compounds in EPA Region 5. Journal of Exposure Analysis and Environmental Epidemiology. 1999;9:381–392. [PubMed: 10554141]
- 22.
- Payne-Sturges DC, et al. Personal exposure meets risk assessment: a comparison of measured and modeled exposures and risks in an urban community. Environmental Health Perspectives. 2004;112:589–598. [PMC free article: PMC1241926] [PubMed: 15064166]
- 23.
- Sax SN, et al. Differences in source emission rates of volatile organic compounds in inner-city residences of New York City and Los Angeles. Journal of Exposure Analysis and Environmental Epidemiology. 2004;14(Suppl. 1):S95–S109. [PubMed: 15118751]
- 24.
- Sexton K, et al. Comparison of personal, indoor, and outdoor exposures to hazardous air pollutants in three urban communities. Environmental Science & Technology. 2004;38:423–430. [PubMed: 14750716]
- 25.
- Van Winkle MR, Scheff PA. Volatile organic compounds, polycyclic aromatic hydrocarbons and elements in the air of ten urban homes. Indoor Air. 2001;11:49–64. [PubMed: 11235231]
- 26.
- Weisel CP, et al. Relationship between indoor, outdoor and personal air (RIOPA). Houston, TX: Health Effects Institute and National Urban Air Toxics Research Center; 2005. (Report No. 130, Part 1)
- 27.
- McDermott MJ, et al. Tetrachloroethylene (PCE, perc) levels in residential dry cleaner buildings in diverse communities in New York City. Environmental Health Perspectives. 2005;113:1336–1343. [PMC free article: PMC1281276] [PubMed: 16203243]
- 28.
- Kirchner S, et al. National dwellings survey: report on air quality in French dwellings, Final Report. Paris: Indoor Air Quality Observatory; 2006.
- 29.
- Jantunen MJ, et al. Air pollution exposure in European cities: the EXPOLIS Study. Kuopio: National Public Health Institute; 1999.
- 30.
- Ohura T, et al. Organic air pollutants inside and outside residences in Shimizu, Japan: levels, sources and risks. Science of the Total Environment. 2006;366:485–499. [PubMed: 16298419]
- 31.
- Report of the nationwide survey of indoor volatile organic chemicals in residential houses, Japan. Tokyo: Ministry of Health and Welfare; 1999. (in Japanese)
- 32.
- Raisanen J, Niemela R, Rosenberg C. Tetrachloroethylene emissions and exposure in dry cleaning. Journal of the Air & Waste Management Association. 2001;51:1671–1675. [PubMed: 15666472]
- 33.
- Moschandreas DJ, Odea DS. Measurement of perchloroethylene indoor air levels caused by fugitive emissions from unvented dry-to-dry dry-cleaning units. Journal of the Air & Waste Management Association. 1995;45:111–115.
- 34.
- Eklund BM, et al. Spatial and temporal variability in VOC levels within a commercial retail building. Indoor Air. 2008;18:365–374. [PubMed: 18636973]
- 35.
- Building Assessment Survey and Evaluation (BASE) Study. Washington, DC: US Environmental Protection Agency; 2008. [2 July 2010]. http://www
.epa.gov/iaq /base/voc_master_list.html. - 36.
- Chao CY, Chan GY. Quantification of indoor VOCs in twenty mechanically ventilated buildings in Hong Kong. Atmospheric Environment. 2001;35:5895–5913.
- 37.
- Loh MM, et al. Measured concentrations of VOCs in several non-residential microenvironments in the United States. Environmental Science & Technology. 2006;4:6903–6911. [PubMed: 17153993]
- 38.
- You XI, et al. Determinants of airborne concentrations of volatile organic compounds in rural areas of Western Canada. Journal of Exposure Analysis and Environmental Epidemiology. 2008;18:117–128. [PubMed: 17327851]
- 39.
- Wallace L. The Total Exposure Assessment Methodology (TEAM) study: summary and analysis. Vol. 1. Washington, DC: US Environmental Protection Agency; 1987. (Report No. EPA/600/6-87/002A)
- 40.
- Wallace LA, Nelson WC, Ziegenfus R. The Los Angeles TEAM study: personal exposures, indoor–outdoor air concentrations, and breath concentrations of 25 volatile organic compounds. Journal of Exposure Analysis and Environmental Epidemiology. 1991;1:157–192. [PubMed: 1824315]
- 41.
- Dallas CE, et al. Use of physiologically based model to predict systemic uptake and respiratory elimination of perchloroethylene. Toxicology and Applied Pharmacology. 1994;128:60–69. [PubMed: 8079355]
- 42.
- Nakai JS, et al. Penetration of chloroform, trichloroethylene, and tetrachloroethylene through human skin. Journal of Toxicology and Environmental Health A. 1999;58:157–170. [PubMed: 10522647]
- 43.
- Poet TS, et al. PBPK modeling of the percutaneous absorption of perchloroethylene from a soil matrix in rats and humans. Toxicological Sciences. 2002;67:17–31. [PubMed: 11961212]
- 44.
- Tetrachloroethylene (PCE). Case studies in environmental medicine. Atlanta, GA: Agency for Toxic Substances and Disease Registry; 2010. [5 July 2010]. www
.atsdr.cdc.gov/csem /pce/pcephysiologic_effects.html. - 45.
- Beliles RP. Concordance across species in the reproductive and developmental toxicity of tetrachloroethylene. Toxicology and Industrial Health. 2002;18:91–106. [PubMed: 12868798]
- 46.
- Dallas CE, et al. Development of a physiologically based model for perchloroethylene using tissue concentration–time data. Toxicology and Applied Pharmacology. 1994;128:50–59. [PubMed: 8079354]
- 47.
- McKernan LT, et al. Biological exposure assessment to tetrachloroethylene for workers in the dry cleaning industry. Environmental Health. 2008;7:12. [PMC free article: PMC2374777] [PubMed: 18412959]
- 48.
- Lash LH, Parker JC. Hepatic and renal toxicities associated with perchloroethylene. Pharmacological Reviews. 2001;53:177–208. [PubMed: 11356983]
- 49.
- Lash LH, et al. Renal toxicity of perchloroethylene and S-(1,2,2-trichlorovinyl)glutathione in rats and mice: sex- and species-dependent differences. Toxicology and Applied Pharmacology. 2002;179:163–171. [PubMed: 11906246]
- 50.
- Philip BK, et al. Impact of repeated exposure on toxicity of perchloroethylene in Swiss Webster mice. Toxicology. 2007;232:1–14. [PubMed: 17267091]
- 51.
- Tetrachloroethylene: assessment of human carcinogenic hazard. Brussels: European Centre for Ecotoxicology and Toxicology of Chemicals; 1990. (ECETOC Technical Report No. 37)
- 52.
- Green T, et al. Perchloroethylene-induced rat kidney tumors: an investigation of the mechanisms involved and their relevance to humans. Toxicology and Applied Pharmacology. 1990;103:77–89. [PubMed: 1969182]
- 53.
- Lash LH, et al. Glutathione conjugation of perchloroethylene in rats and mice in vitro: sex-, species-, and tissue-dependent differences. Toxicology and Applied Pharmacology. 1998;150:49–57. [PubMed: 9630452]
- 54.
- Völkel W, et al. Biotransformation of perchloroethene: dose-dependent excretion of trichloroacetic acid, dichloroacetic acid, and N-acetyl-S-(trichlorovinyl)-L-cysteine in rats and humans after inhalation. Toxicology and Applied Pharmacology. 1998;153:20–27. [PubMed: 9875296]
- 55.
- Ohtsuki T, et al. Limited capacity of humans to metabolize tetrachloroethylene. International Archives of Occupational and Environmental Health. 1983;51:381–390. [PubMed: 6862652]
- 56.
- Bois FY, et al. Population toxicokinetics of tetrachloroethylene. Archives of Toxicology. 1996;70:347–355. [PubMed: 8975633]
- 57.
- Chiu WA, et al. Toxicokinetics of inhaled trichloroethylene and tetrachloroethylene in humans at 1 ppm: empirical results and comparisons with previous studies. Toxicological Sciences. 2007;95:23–36. [PubMed: 17032701]
- 58.
- Byczkowski JZ, et al. Computer simulation of the lactational transfer of tetrachloroethylene in rats using a physiologically based model. Toxicology and Applied Pharmacology. 1994;125:228–236. [PubMed: 8171430]
- 59.
- Hamada T, Tanaka H. Transfer of methyl chloroform, trichloroethylene and tetrachloroethylene to milk, tissues and expired air following intraruminal or oral administration in lactating goats and milk-fed kids. Environmental Pollution. 1995;87:313–318. [PubMed: 15091581]
- 60.
- Schreiber JS. Predicted infant exposure to tetrachloroethylene in human breastmilk. Risk Analysis. 1993;13:515–524. [PubMed: 8259441]
- 61.
- Gobba F, et al. Perchloroethylene in alveolar air, blood, and urine as biologic indices of low-level exposure. Journal of Occupational and Environmental Medicine. 2003;45:1152–1157. [PubMed: 14610396]
- 62.
- Lauwerys R, et al. Health surveillance of workers exposed to tetrachloroethylene in dry-cleaning shops. International Archives of Occupational and Environmental Health. 1983;52:69–77. [PubMed: 6874093]
- 63.
- Furuki K, et al. Monitoring of occupational exposure to tetrachloroethene by analysis for unmetabolized tetrachloroethene in blood and urine in comparison with urinalysis for trichloroacetic acid. International Archives of Occupational and Environmental Health. 2000;73:221–227. [PubMed: 10877027]
- 64.
- Chen CW, Blancato JN. Pharmacokinetics in risk assessment – drinking water and health. Vol. 8. Washington DC: National Academy Press; 1987. Role of pharmacokinetic modeling in risk assessment: perchloroethylene as an example.
- 65.
- Bogen KT, McKone TE. Linking indoor air and pharmacokinetic models to assess tetrachloroethylene risk. Risk Analysis. 1988;8:509–552. [PubMed: 3244858]
- 66.
- Ward RC, et al. Pharmacokinetics of tetrachloroethylene. Toxicology and Applied Pharmacology. 1988;93:108–117. [PubMed: 3353997]
- 67.
- Koizumi A. Potential of physiologically based pharmacokinetics to amalgamate kinetic data of trichloroethylene and tetrachloroethylene obtained in rats and man. British Journal of Industrial Medicine. 1989;46:239–249. [PMC free article: PMC1009763] [PubMed: 2713280]
- 68.
- Dallas CE, et al. Use of tissue distribution data from rats and dogs to determine species differences in input parameters for a physiological model for perchloroethylene. Environmental Research. 1994;67:54–67. [PubMed: 7925194]
- 69.
- Dallas CE, et al. Physiologically based pharmacokinetic model useful in prediction of the influence of species, dose, and exposure route of perchloroethylene pharmacokinetics. Journal of Toxicology and Environmental Health. 1995;44:301–317. [PubMed: 7897693]
- 70.
- Jang JY, Droz PO. Ethnic differences in biological monitoring of several organic solvents. II. A simulation study with a physiologically based pharmacokinetic model. International Archives of Occupational and Environmental Health. 1997;70:41–50. [PubMed: 9258706]
- 71.
- Gearhart JM, et al. Variability of physiologically based pharmacokinetic (PBPK) model parameters and their effects on PBPK model predictions in a risk assessment for perchloroethylene (PCE). Toxicology Letters. 1993;68:131–144. [PubMed: 8516760]
- 72.
- Reitz RH, et al. In vivo and in vitro studies of perchloroethylene metabolism for physiologically based pharmacokinetic modeling in rats, mice, and humans. Toxicology and Applied Pharmacology. 1996;136:289–306. [PubMed: 8619237]
- 73.
- Dobrev ID, Andersen ME, Yang RS. Assessing interaction thresholds for trichloroethylene in combination with tetrachloroethylene and 1,1,1-trichloroethane using gas uptake studies and PBPK modeling. Archives of Toxicology. 2001;75:134–144. [PubMed: 11409535]
- 74.
- Loizou GD. The application of physiologically based pharmacokinetic modelling in the analysis of occupational exposure to perchloroethylene. Toxicology Letters. 2001;124:59–69. [PubMed: 11684358]
- 75.
- Poet TS, et al. PBPK modeling of the percutaneous absorption of perchloroethylene from a soil matrix in rats and humans. Toxicological Sciences. 2002;67:17–31. [PubMed: 11961212]
- 76.
- Dobrev ID, Andersen ME, Yang RS. In silico toxicology: simulating interaction thresholds for human exposure to mixtures of trichloroethylene, tetrachloroethylene, and 1,1,1-trichloroethane. Environmental Health Perspectives. 2002;110:1031–1039. [PMC free article: PMC1241030] [PubMed: 12361929]
- 77.
- Clewell HJ, et al. Evaluation of physiologically based pharmacokinetic models in risk assessment: an example with perchloroethylene. Critical Reviews in Toxicology. 2005;35:413–433. [PubMed: 16097137]
- 78.
- Chiu WA, Bois FY. Revisiting the population toxicokinetics of tetrachloroethylene. Archives of Toxicology. 2006;80:382–385. Erratum. Archives of Toxicology, 2006, 80:386. [PubMed: 16474962]
- 79.
- Covington TR, et al. The use of Markov chain Monte Carlo uncertainty analysis to support a Public Health Goal for perchloroethylene. Regulatory Toxicology and Pharmacology. 2007;47:1–18. [PubMed: 16901594]
- 80.
- Ramsey JC, Andersen ME. A physiologically based description of the inhalation pharmacokinetics of styrene in rats and humans. Toxicology and Applied Pharmacology. 1984;73:159–175. [PubMed: 6710512]
- 81.
- Hattis D, et al. Uncertainties in pharmacokinetic modelling for perchloroethylene. I. Comparison of model structure, parameters, and predictions for low-dose metabolism rates for models derived by different authors. Risk Analysis. 1990;10:449–458. [PubMed: 2236748]
- 82.
- Bois FY, et al. Precision and sensitivity of pharmacokinetic models for cancer risk assessment: tetrachloroethylene in mice, rats and humans. Toxicology and Applied Pharmacology. 1990;102:300–315. [PubMed: 2300971]
- 83.
- Kjellstrand P, et al. Perchloroethylene: effects on body and organ weights and plasma butyrylcholinesterase activity in mice. Acta Pharmcologica et Toxicologica. 1984;54:414–424. [PubMed: 6464786]
- 84.
- Technical report on the toxicology carcinogenesis studies of tetrachloroethylene (perchloroethylene) in F344/N rats and B6C3F1 mice (inhalation studies). Research Triangle Park, NC: National Toxicology Program; 1986. (NTP Technical Report No. 311) [PubMed: 12748718]
- 85.
- Suzaki H, Aoki A, Nomura Y. Regeneration of olfactory mucosa in mice after inhalation exposure to perchloroethylene. Journal for Otorhinolaryngology and its Related Species. 1997;59:91–96. [PubMed: 9166878]
- 86.
- Wang S, et al. Perchloroethylene-induced reduction of glial and neuronal cell marker proteins in rat brain. Pharmacology and Toxicology. 1993;72:273–278. [PubMed: 8372046]
- 87.
- Seidel HJ, et al. Hematological toxicity of tetrachloroethylene in mice. Archives of Toxicology. 1992;66:228–230. [PubMed: 1497490]
- 88.
- Chen HH, Chan MH, Fu SH. Behavioural effects of tetrachloroethylene exposure in rats: acute and subchronic studies. Toxicology. 2002;170:201–209. [PubMed: 11788157]
- 89.
- Oshiro WM, Krantz QT, Bushnell PJ. Characterization of the effects of inhaled perchloroethylene on sustained attention in rats performing a visual signal detection task. Neurotoxicology and Teratology. 2008;30:167–174. [PubMed: 18299185]
- 90.
- Saillenfait AM, Langonné I, Sabaté JP. Developmental toxicity of trichloroethylene, tetrachloroethylene and four of their metabolites in rat whole embryo culture. Archives of Toxicology. 1995;70(2):71–82. [PubMed: 8773178]
- 91.
- Fredriksson A, Danielsson BR, Eriksson P. Altered behaviour in adult mice orally exposed to tri- and tetrachloroethylene as neonates. Toxicology Letters. 1993;66:13–19. [PubMed: 8427017]
- 92.
- Carney EW, et al. Developmental toxicity studies in Crl:CD (SD) rats following inhalation exposure to trichloroethylene and perchloroethylene. Birth Defects Research (Part B). 2006;77:405–412. [PubMed: 17066414]
- 93.
- Chen HH, et al. Effects of trichloroethylene and perchloroethylene on muscle contractile responses and epithelial prostaglandin release and acetylcholinesterase activity in swine trachea. Toxicological Sciences. 2005;83:149–154. [PubMed: 15496499]
- 94.
- Technical report on the bioassay of tetrachloroethylene (CAS No. 127-18-4) for possible carcinogenesis. Bethesda, MD: Department of Health, Education and Welfare; 1977. (DHEW Publication No. 77-813; National Cancer Institute Technical Report No. 13)
- 95.
- Ishmael J, Dugard PH. A review of perchloroethylene and rat mononuclear cell leukemia. Regulatory Toxicology and Pharmacology. 2006;45:178–184. [PubMed: 16684583]
- 96.
- Dry cleaning, some chlorinated solvents and other industrial chemicals. Lyon: International Agency for Research on Cancer; 1997. (IARC Monographs on the Evaluation of Carcinogenic Risks to Humans, Vol. 63)
- 97.
- Murakami K, Horikawa K. The induction of micronuclei in mice hepatocytes and reticulocytes by tetrachloroethylene. Chemosphere. 1995;31:3733–3739. [PubMed: 8528655]
- 98.
- Giovannini L, et al. Effect of ethanol chronic use on hepatotoxicity in rats exposed to tetrachloroethylene. International Journal of Tissue Reactions. 1992;24:281–285. [PubMed: 1306529]
- 99.
- Dobrev ID, Andersen ME, Yang RS. In silico toxicology: simulating interaction thresholds for human exposure to mixtures of trichloroethylene, tetrachloroethylene, and 1,1,1-trichloroethane. Environmental Health Perspectives. 2002;110:1031–1039. [PMC free article: PMC1241030] [PubMed: 12361929]
- 100.
- Köppel C, et al. Acute tetrachloroethylene poisoning – blood elimination kinetics during hyperventilation therapy. Journal of Toxicology, Clinical Toxicology. 1985;23:103–115. [PubMed: 4057308]
- 101.
- Choi YH, et al. ARF requiring hemodialysis after accidental perchloroethylene ingestion. American Journal of Kidney Diseases. 2003;41:E11. [PubMed: 12613004]
- 102.
- Levine B, et al. A tetrachloroethylene fatality. Journal of Forensic Sciences. 1981;26:206–209. [PubMed: 7205186]
- 103.
- Garnier R, et al. Coin-operated dry cleaning machines may be responsible for acute tetrachloroethylene poisoning: report of 26 cases including one death. Journal of Toxicology, Clinical Toxicology. 1996;34:191–197. [PubMed: 8618253]
- 104.
- Gaillard Y, Billault F, Pépin G. Tetrachloroethylene fatality: case report and simple gas chromatographic determination in blood and tissues. Forensic Science International. 1995;76:161–168. [PubMed: 8566918]
- 105.
- Lukaszewski T. Acute tetrachloroethylene fatality. Clinical Toxicology. 1979;15:411–415. [PubMed: 540489]
- 106.
- Dehon B, et al. Tetrachloroethylene and trichloroethylene fatality: case report and simple headspace SPME-capillary gas chromatographic determination in tissues. Journal of Analytical Toxicology. 2000;24:22–26. [PubMed: 10654565]
- 107.
- Hake CL, Stewart RD. Human exposure to tetrachloroethylene: inhalation and skin contact. Environmental Health Perspectives. 1977;21:231–238. [PMC free article: PMC1475318] [PubMed: 612448]
- 108.
- Altmann L, et al. Neurophysiological and psychological measurements reveal effects of acute low-level organic solvent exposure in humans. International Archives of Occupational and Environmental Health. 1990;62:493–499. [PubMed: 2289821]
- 109.
- Seeber A. Neurobehavioral toxicology of long-term exposure to tetrachloroethylene. Neurotoxicology and Teratology. 1990;11:579–583. [PubMed: 2626148]
- 110.
- Ferroni C, et al. Neurobehavioral and neuroendocrine effects of occupational exposure to perchloroethylene. Neurotoxicology. 1992;13:243–248. [PubMed: 1508425]
- 111.
- Nakatsuka H, et al. Absence of blue–yellow color vision loss among workers exposed to toluene or tetrachloroethylene mostly at levels below occupational exposure limits. International Archives of Occupational and Environmental Health. 1992;64:113–117. [PubMed: 1399020]
- 112.
- Cavalleri A, et al. Perchloroethylene exposure can induce color vision loss. Neuroscience Letters. 1994;179:162–166. [PubMed: 7845613]
- 113.
- Gobba F, et al. Two-year evolution of perchloroethylene-induced color-vision loss. Archives of Environmental Health. 1998;53:196–198. [PubMed: 9814715]
- 114.
- Onofrj M, et al. Optic neuritis with residual tunnel vision in perchloroethylene toxicity. Journal of Toxicology, Clinical Toxicology. 1998;36:603–607. [PubMed: 9776966]
- 115.
- Perrin MC, et al. Tetrachloroethylene exposure and risk of schizophrenia: offspring of dry cleaners in a population birth cohort, preliminary findings. Schizophrenia Research. 2007;90:251–254. [PMC free article: PMC2739584] [PubMed: 17113267]
- 116.
- Storm JE, Mazor KA. Update of residential tetrachloroethylene exposure and decreases in visual contrast sensitivity. Environmental Health Perspectives. 2004;112:A862–A864. author reply A864–A865. Erratum. Environmental Health Perspectives, 2004, 112:A980. [PMC free article: PMC1247642] [PubMed: 15531411]
- 117.
- Brodkin CA, et al. Hepatic ultrasonic changes in workers exposed to perchloroethylene. Occupational and Environmental Medicine. 1995;52:679–685. [PMC free article: PMC1128334] [PubMed: 7489059]
- 118.
- Mutti A, et al. Nephropathies and exposure to perchloroethylene in dry-cleaners. Lancet. 1992;340:189–193. [PubMed: 1353133]
- 119.
- Verplanke AJ, Leummens MH, Herber RF. Occupational exposure to tetrachloroethene and its effects on the kidneys. Journal of Occupational and Environmental Medicine. 1999;41:11–16. [PubMed: 9924715]
- 120.
- Franchini I, et al. Early indicators of renal damage in workers exposed to organic solvents. International Archives of Occupational and Environmental Health. 1983;52:1–9. [PubMed: 6603422]
- 121.
- Sonnenfeld N, Hertz-Picciotto I, Kaye WE. Tetrachloroethylene in drinking water and birth outcomes at the US Marine Corps Base at Camp Lejeune, North Carolina. American Journal of Epidemiology. 2001;154:902–908. [PubMed: 11700244]
- 122.
- Bove FJ, et al. Public drinking water contamination and birth outcomes. American Journal of Epidemiology. 1995;141:850–862. [PubMed: 7717362]
- 123.
- Chen ATL, Sever LE. Comment on: Public drinking water contamination and birth outcomes. American Journal of Epidemiology. 1996;143:1179–1180. [PubMed: 8633613]
- 124.
- Mills JL, et al. Homocysteine metabolism in pregnancies complicated by neural-tube defects. Lancet. 1995;345:149–151. [PubMed: 7741859]
- 125.
- Janulewicz PA, et al. Risk of learning and behavioral disorders following prenatal and early postnatal exposure to tetrachloroethylene (PCE)-contaminated drinking water. Neurotoxicology and Teratology. 2008;30:175–185. [PMC free article: PMC2494864] [PubMed: 18353612]
- 126.
- Aschengrau A, et al. Prenatal exposure to tetrachloroethylene-contaminated drinking water and the risk of adverse birth outcomes. Environmental Health Perspectives. 2008;116:814–820. [PMC free article: PMC2430239] [PubMed: 18560539]
- 127.
- Doyle P, et al. Spontaneous abortion in dry cleaning workers potentially exposed to perchloroethylene. Occupational and Environmental Medicine. 1997;54:848–853. [PMC free article: PMC1128964] [PubMed: 9470891]
- 128.
- Olsen J, et al. Low birthweight, congenital malformations, and spontaneous abortions among dry-cleaning workers in Scandinavia. Scandinavian Journal of Work and Environmental Health. 1990;16:163–168. [PubMed: 2143312]
- 129.
- Kyyrönen P, et al. Spontaneous abortions and congenital malformations among women exposed to tetrachloroethylene in dry cleaning. Journal of Epidemiology and Community Health. 1989;43:346–351. [PMC free article: PMC1052872] [PubMed: 2614324]
- 130.
- McDonald AD, et al. Spontaneous abortion and occupation. Journal of Occupational Medicine. 1986;28:1232–1238. [PubMed: 3806263]
- 131.
- Bosco MG, Figá-Talamanca I, Salerno S. Health and reproductive status of 24 female workers in dry cleaning shops. International Archives of Occupational and Environmental Health. 1987;59:295–301. [PubMed: 3570493]
- 132.
- Ahlborg G Jr. Pregnancy outcome among women working in laundries and dry-cleaning shops using tetrachloroethylene. American Journal of Industrial Medicine. 1990;17:567–575. [PubMed: 2337082]
- 133.
- Sallmén M, et al. Reduced fertility among women exposed to organic solvents. American Journal of Industrial Medicine. 1995;27:699–713. [PubMed: 7611306]
- 134.
- Agency for Toxic Substances and Disease Registry (ATSDR). Volatile organic compounds in drinking water and adverse pregnancy outcomes: United States Marine Corps Base, Camp Lejeune, North Carolina. Atlanta, GA: US Department of Health and Human Services; 1998.
- 135.
- Tetrachloroethylene (perchloroethylene). Research Triangle Park, NC: National Toxicology Program; 2002. (Report on Carcinogens, 10th ed., pp. 226–228)
- 136.
- Toraason M, et al. Effect of perchloroethylene, smoking, and race on oxidative DNA damage in female dry cleaners. Mutation Research. 2003;539:9–18. [PubMed: 12948810]
- 137.
- Diodovich C, et al. Sensitivity of human cord blood cells to tetrachloroethylene: cellular and molecular endpoints. Archives of Toxicology. 2005;79:508–514. [PubMed: 16010555]
- 138.
- Aschengrau A, Paulu C, Ozonoff D. Tetrachloroethylene-contaminated drinking water and the risk of breast cancer. Environmental Health Perspectives. 1998;106(Suppl. 4):947–953. [PMC free article: PMC1533339] [PubMed: 9703477]
- 139.
- Aschengrau A, Rogers S, Ozonoff D. Perchloroethylene-contaminated drinking water and the risk of breast cancer: additional results from Cape Cod, Massachusetts, USA. Environmental Health Perspectives. 2003;111:167–173. [PMC free article: PMC1241345] [PubMed: 12573900]
- 140.
- Vieira V, Aschengrau A, Ozonoff D. Impact of tetrachloroethylene-contaminated drinking water on the risk of breast cancer: using a dose model to assess exposure in a case-control study. Environmental Health. 2005;4:3. [PMC free article: PMC554766] [PubMed: 15733317]
- 141.
- Paulu C, Aschengrau A, Ozonoff D. Tetrachloroethylene-contaminated drinking water in Massachusetts and the risk of colon-rectum, lung, and other cancers. Environmental Health Perspectives. 1999;107:265–271. [PMC free article: PMC1566514] [PubMed: 10090704]
- 142.
- Mundt KA, Birk T, Burch MT. Critical review of the epidemiological literature on occupational exposure to perchloroethylene and cancer. International Archives of Occupational and Environmental Health. 2003;76:473–491. [PubMed: 12898270]
- 143.
- Ruder AM. Potential health effects of occupational chlorinated solvent exposure. Annals of the New York Academy of Sciences. 2006;1076:207–227. [PubMed: 17119204]
- 144.
- European Commission. Human exposure characterisation of chemical substances, quantification of exposure routes. Ispra: Physical and Chemical Exposure Unit Joint Research Centre; 2005.
- 145.
- Tetrachloroethylene (perchloroethylene). Air Toxics Web Site. Washington, DC: US Environmental Protection Agency; 2000. [17 July 2010]. http://www
.epa.gov/ttn /atw/hlthef/tet-ethy.html. - 146.
- Department of Health. 1996 Annual report of the Committees on Toxicity, Mutagenicity and Carcinogenicity of Chemicals in Food, Consumer Products and the Environment. London: The Stationery Office; 1997.
- 147.
- NIOSH pocket guide to chemical hazards. Tetrachloroethylene. Atlanta, GA: National Institute for Occupational Safety and Health; 2005. (NIOSH Publication 2005-149)
- 148.
- Air quality guidelines for Europe. 2nd ed. Copenhagen: WHO Regional Office for Europe; 2000. Tetrachloroethylene. (WHO Regional Publications, European Series, No. 91)
Footnotes
- 1
This figure was calculated and found to be 0.24 mg/m3 using the information provided by ATSDR. This may be due to a different conversion factor for converting ppm to mg/m3 and rounding during the calculation.
- Tetrachloroethylene - WHO Guidelines for Indoor Air Quality: Selected PollutantsTetrachloroethylene - WHO Guidelines for Indoor Air Quality: Selected Pollutants
Your browsing activity is empty.
Activity recording is turned off.
See more...