NCBI Bookshelf. A service of the National Library of Medicine, National Institutes of Health.
Madame Curie Bioscience Database [Internet]. Austin (TX): Landes Bioscience; 2000-2013.
The cGMP-gated channel of rod photoreceptors plays a key role in phototransduction by controlling the flow of cations into the outer segment in response to light-induced changes in cGMP. The channel is a heterotetramer composed of α-subunits required for channel activity and β-subunits that are important in modulating the activity of channel. Earlier studies have shown that exogenous calmodulin binds to the β-subunit of the channel and modulates the sensitivity of the channel for cGMP in a calcium-dependent manner. In addition unidentified Ca2+-dependent endogenous proteins have been reported to modulate the activity of the frog rod channel. In this paper, we investigated whether endogenous calmodulin and other Ca2+ binding proteins interact with and modulate the cGMP-gated channel in bovine rod outer segments. Using immunoaffinity techniques in conjunction with ion flux assays, we show that endogenous calmodulin, but not other Ca2+-dependent proteins, binds and modulates the rod cGMP-gated channel in bovine rod outer segments. We also show that the β-subunit of the channel is phosphorylated by endogenous and exogenous casein kinase 2. This posttranslational modification, however, does not alter the sensitivity of the channel for cGMP.
Introduction
The cyclic nucleotide-gated (CNG) channel of the rod photoreceptor cell plays a key role in phototransduction by controlling the flow of Na and Ca2+ into the rod outer segment (ROS) in response to light-induced changes in intracellular cGMP concentration. In the dark, a significant number of CNG channels are maintained in their open state by the reversible binding of cGMP to the channel1,2(Fig. 1). This allows Na and Ca2+ to enter the outer segment, establishing a dark current between the outer and inner segment. Under these conditions, the rod photoreceptor is in its depolarized state and there is a continuous release of glutamate transmitter at the rod synapse. Low intracellular Na concentration is maintained by the active transport of Na from the cell through a Na/K-ATPase in the plasma membrane of the rod inner segment. The Ca2+ concentration in the ROS is kept at 400-500 nM by the balanced extrusion of Ca2+ from the ROS by the Na/Ca-K exchanger.
Photobleaching of rhodopsin in the disk membrane leads to the activation of the G-protein-mediated visual cascade that culminates in a decrease in cGMP concentration and the closure of the CNG channel to the influx of Na and Ca2+ (Fig. 1).35 Suppression of the dark current and the corresponding hyperpolarization of the rod cell inhibits the release of glutamate at the synapse. Closure of the CNG channel also decreases the intracellular Ca2+ concentration to below 100 nM as the Na/Ca-K exchanger continues to extrude Ca2+from the ROS.6,7
The rod photoreceptor cell returns to its dark state following photoexcitation through a series of biochemical reactions.1,8 Photoexcited rhodopsin is inactivated by rhodopsin kinase catalyzed phosphorylation followed by the binding of arrestin. The G-protein-coupled cascade is inactivated by RGS9 protein mediated hydrolysis of GTP to GDP bound to transducin.9 This results in the return of phosphodiesterase (PDE) to its prebleach, basal activity and restoration of transducin to its inactive state (Fig. 1). Finally, the cGMP concentration is restored to its dark level through the Ca2+-dependent activation of guanylate cyclase (10). The rise in cGMP concentration reopens the CNG channels and returns the rod cell to its depolarized state.
The decrease in intracellular Ca2+ following illumination initiates multiple negative feedback mechanisms that contribute to light adaptation and photorecovery.1,8,11 These mechanisms typically involve Ca2+-binding proteins that sense and respond to small changes in free intracellular Ca2+ levels.12,13 Modulation of guanylate cyclase by guanylate cyclase-activating proteins, known as GCAPs, is the principal Ca2+-dependent mechanism.14,16 Regulation of the lifetime of PDE presumably through the modulation of rhodopsin kinase by recoverin, also known as S-modulin, has also been suggested to play a role in light adaptation under certain conditions.1,17 Finally, the sensitivity of the CNG channel to cGMP is altered by the Ca2+-dependent binding of calmodulin (CaM) to the channel.18 Other, as yet unidentified, Ca2+-dependent factors, have also been implicated in the control of rod channel sensitivity.19,20
In this chapter, the structural features of the rod CNG channel and its interaction with ROS proteins is discussed. Biochemical experiments designed to determine if endogeneous, as well as exogeneous, CaM binds to and modulates the rod CNG channel in ROS are reported. Finally, studies to assess the regulation of the channel by other Ca-binding factors and phosphorylation are described.
Rod CNG Channel Structural Features
The structural and functional properties of CNG channels have been the subject of a number of recent reviews.21,23,24,25 Briefly, the rod channel is a heterotetrameric complex consisting of two types of subunits designated as α or CNGA1 and β or CNGB1 subunits.26,29They are 30% identical in sequence and contain similar structural features including six transmembrane segments (S1S6), a pore region of approximately 25 amino acids situated between S5 and S6 segments, and a cyclic nucleotide binding domain near the C terminus (Fig. 2A).
The α subunit is essential for channel activity since it self-assembles into a functional cGMP-gated channel when expressed in Xenopus oocytes or HEK 293 cells.26,30 In contrast, the β-subunit itself fails to form a functional channel when individually expressed in heterologous cells.27,28 Coexpression of the α and β subunits, however, produces a channel that has essentially identical physiological and pharmacological properties (cyclic nucleotide dependence, rapid opening-closing or flickering behavior, selective ion permeability, divalent ion blockage, l-cis-diltiazem inhibition and Ca-calmodulin modulation) of the native rod channel, indicating that the β-subunit is important in fine tuning and modulating the cGMP-dependent ion conducting activity of the channel.27,28,31
The α-subunit has several structural features not found in the β-subunit. An oligosaccharide chain is linked to an asparagine residue located between the S5 transmembrane segment and the pore region.32The pore region harbors a negatively charged glutamate residue that binds extracellular Ca2+ and Mg2+.33,34 This reduces the current through the channel, a property that contributes to low background noise in rod cells. The glutamate residue within the pore region of the α-subunit is replaced with a neutral glycine residue in the β-subunit. Finally, the rod α-subunit undergoes a photoreceptor-specific, posttranslational cleavage that removes the N-terminal 92 amino acids.35 The role of this protein modification is not known at the present time.
The bovine rod β-subunit is unique in that it contains a large cytoplasmic N-terminal region (592 amino acids in the bovine β-subunit) known as the GARP part28(Fig. 2A). GARP, in addition to being part of the β-subunit, is also expressed in ROS as two alternatively spliced variants referred to as GARP1 (also known as fGARP) and GARP2 (also known as tGARP).36,38 GARP1 is identical in sequence to the GARP part of the channel except for an additional 19-amino acid extension at the C terminus. GARP2, the more abundant variant, is a truncated form of GARP1 consisting of the N-terminal 291 amino acids and an 8-amino acid C-terminal extension. The GARP variants are characterized by a high content of glutamate and proline residues that affect their migration on SDS polyacrylamide gels. Recently, four proline-rich repeat regions have been identified within the N-terminal half of the GARP proteins.38 The GARP part of the β-subunit does not influence the rod channel activity.28 Instead, several recent studies indicate that this region plays a role in protein-protein interactions within ROS.38,39 The β-subunit of the CNG channel also contains a binding site for CaM as discussed in detail below.
The rod CNG channel is known to exist in membranes as tetramer. The stoichiometry and arrangement of subunits of the native channel of ROS remains to be determined. However, recent heterologous expression studies suggest that the channel consists of two α and two β subunits with identical subunits arranged diagonal to each other (Fig 2B).40 By analogy with the structure of the K channel of Streptomyces lividans,41 the four subunits are thought to form a central cavity through which cations flow. This cavity is most likely lined by the S5 and S6 transmembrane segments of the α- and β-subunits (Fig 2). The pore region of each subunit extends into the central cavity, where together they function as the gate and selectivity filter for the flow of ions through the membrane.
The rod channel is cooperatively activated by cGMP with a Hill coefficient greater than 3 and a K1/2 of 2050 μM cGMP (21, 22). The channel has a relatively high probability of being in its open state when 34 cGMP molecules are bound.42
Regulation of the Rod Channel: Exogenous Calmodulin (CaM) Regulates the Rod Channel Sensitivity for cGMP
CaM is a highly conserved, 148-amino acid Ca2+-binding protein that regulates a wide variety of enzymes, channels, transporters, and cytoskeletal proteins in eukaryotic cells. It has a dumbbell-like shape with two globular lobes connected by a seven-turn α helix. Each lobe has two high-affinity Ca2+ binding sites that are formed by helix-loop-helix motifs known as EF hands. The binding of Ca2+ to these sites induces a protein conformational change enabling CaM to interact and regulate target proteins. The site of interaction on the target protein typically consists of an amphipathic helix.
Modulation of the rod CNG channel by exogeneous CaM was first demonstrated using ion flux assays in ROS vesicle preparations.18 The sensitivity of the channel for cGMP was decreased when CaM was added in the presence of excess Ca2+. This was reflected in an increase in the apparent K1/2 for cGMP from 19 μM without CaM to 33 μM with CaM. The cooperativity of the channel for cGMP was not affected by the presence of CaM. Modulation of the sensitivity of the rod channel for cGMP by exogeneous CaM has been reproduced in several different experimental systems including purified and reconstituted channel preparations, excised patches from ROS, and the heteromeric rod CNG channel expressed in HEK 293 cells.19,28,31,43,44,45 CaM regulation of the channel is Ca2+-dependent, occurring over the physiological relevant Ca2+ concentration range of 50 to 300 nM Ca2+.43,44 The olfactory channel is also modulated by CaM, but to a significantly larger extent. CaM decreases the cyclic nucleotide sensitivity of the olfactory channel by 1020-fold.46 This mechanism plays a dominant role in olfactory adaptation. The binding site for CaM resides on the β- subunit of the rod channel. This was initially determined using gel overlay methods and functional analysis of homomeric and heteromeric channels.18,31 More recently, Weitz et al47 and Grunwald et al48 mapped the location of two CaM binding sites within the β-subunit. One site was localized to a 15-amino acid segment just before the first membrane-spanning segment (S1) of the β-subunit. Deletion of this site abolished binding and modulation of the channel by CaM. A second site was found downstream of the cyclic nucleotide binding domain. Deletion of this site, however, had little effect on CaM modulation. The sites are unusual since they do not harbor an amphipathic helical binding motif characteristic of most CaM binding proteins. The binding affinity of CaM for these individual target sites is 23 orders of magnitude lower than the affinity of CaM for the intact channel, estimated to be approximately 15 nM.43,44 This suggests that the conformation of the protein is important in establishing a high-affinity CaM-binding site.
Endogeneous CaM is the Major Ca2+ Regulator of the Rod Channel
In previous studies, exogenous CaM was added to samples to demonstrate binding and modulation of the rod channel. In order to further evaluate the potential role of this regulatory mechanism in rod photoreceptor function, we have used immunoprecipitation techniques to examine whether CaM in ROS interacts with the rod channel in a Ca2+-dependent manner. In these studies, ROS were washed in hypotonic buffer (10 μM HEPES, pH 7.4, 1 μM DTT) containing either 1 μM CaCl2 or μM EDTA and the membranes were solubilized in CHAPS buffer (10 μM HEPES, pH 7.4, 18 μM CHAPS, 150 μM KCl, 1μM CaCl2). The rod CNG channel was then immunoprecipitated with an antichannel (α- subunit) monoclonal antibody (PMc 1D1) immobilized on Sepharose beads. Coprecipitation of CaM with the channel was analyzed by Western blotting using a CaM monoclonal antibody. As shown in Fig. 3, CaM coprecipitates with the rod channel from ROS washed in the presence of Ca2+, but not in the absence of Ca2+ i.e. EDTA-treated ROS. This indicates that endogeneous CaM associates with the rod channel in the presence of Ca2+ and dissociates when Ca2+ is removed.
In a previous study, Gordon et al19reported that frog ROS contain an unidentified, endogenous Ca2+-binding protein that regulates the channel in a similar way as CaM, but over a different range of Ca2+ concentrations. From these studies, they concluded that this endogenous Ca2+-binding protein associates with the channel at the same site as CaM.
To determine if bovine ROS contain a related endogeneous Ca2+-binding protein, we investigated the interaction of the channel with CaM-Sepharose matrix in the presence and absence of endogeneous CaM. ROS were washed in hypotonic buffer containing 2 μM EDTA to remove endogenous CaM bound to the channel. The ROS membranes were then solubilized in CHAPS buffer and incubated with HEPES (buffer control), supernatant from hypotonically lysed ROS containing endogenous CaM, and supernatant from hypotonically lysed ROS in which CaM was removed by immunoprecipitation with an antiCaM antibody (Fig. 4A). As shown in Figure 4B, the channel derived from solubilized ROS treated with CaM-depleted soluble extract quantitatively bound to the CaM-Sepharose matrix. In contrast, the channel from solubilized ROS treated with extract containing endogenous CaM did not bind to the CaM-Sepharose matrix. These data indicate that bovine ROS extracts do not contain an endogenous protein that effectively competes with CaM for the same binding site on the channel.
The ability of endogenous CaM or another factor to modulate the sensitivity of the channel for cGMP was investigated using ion flux assays. In these studies, the channel from ROS was reconstituted into Ca2+-containing lipid vesicles as previously described.49 Cyclic GMP-dependent efflux of Ca2+ was measured in the presence and absence of soluble extracts from hypotonically lysed ROS. Fig. 5A shows cGMP-dependent efflux of Ca2+ as a function of time and Fig. 5B shows the normalized rate of efflux as a function of cGMP concentration. A decrease in sensitivity of the channel for cGMP is observed only for vesicles treated with soluble extract containing endogenous CaM. Vesicles treated with soluble extract depleted of CaM exhibited the same sensitivity to cGMP as the buffer control. These results indicate that endogenous CaM modulates the rod channel to the same degree as exogenously added CaM. Furthermore, soluble extracts from bovine ROS do not contain other factors that modulate the sensitivity of the channel for cGMP.
Phosphorylation of the Channel
A number of ROS proteins that function in phototransduction undergo kinase-catalyzed phosphorylation reactions. These include rhodopsin, guanylate cyclase, rhodopsin kinase, RGS9, transducin among others. In some instances, phosphorylation directly regulates the activity of these proteins such as in the case of rhodopsin kinase-catalyzed phosphorylation of rhodopsin.50
Several reports have suggested that the activity of the rod CNG channel is also affected by phosphorylation. Using patch-clamp techniques, Gordon et al51 showed that serine/threonine inhibitors or exogenous phosphatase alter the sensitivity of the channel for cGMP in frog ROS. The native and expressed rod channel α-subunit is also modulated by tyrosine kinase and phosphatase inhibitors.52,53 Regulation of the channel by tyrosine phosphorylation appears to occur through a signal transduction pathway initiated by the binding of insulin-like growth factors to surface rod cells.54Although these studies suggest that the channel can be regulated by phosphorylation reactions, direct phosphorylation of the channel was not determined in these studies.
We have carried out a number of studies to determine if the rod CNG channel can be phosphorylated by an endogenous protein kinase in ROS. In one series of experiments, ROS were hypotonically lysed in 0.01 M HEPES buffer and the ROS membranes were separated from the soluble extract by centrifugation. The ROS membranes were resuspended in HEPES buffer or ROS-soluble extract, and the phosphorylation reaction was initiated by the addition of [γ-32P]ATP. After 15 min at 30°C, the membranes were washed in HEPES buffer, and the channel was isolated on a PMc 1D1 antichannel-Sepharose affinity matrix. As shown in Fig. 6, the β-subunit, but not the α-subunit, of the rod channel was phosphorylated by an endogenous kinase. The extent of of phosphorylation was significantly greater when the reaction was carried out in the presence of ROS soluble extract. This suggests that the protein kinase that catalyzed the phosphorylation of the β-subunit exists primarily as a soluble protein in ROS.
We examined the effect of a number of factors, known to be important in phototransduction, on channel phosphorylation. The extent of phosphorylation was independent of the light/dark state of ROS or the presence of up to 2 μM Ca2+ or EDTA, 100 μM phorbol ester with and without Ca2+, 200 μM cGMP or BrcGMP, 100 μM cAMP or CaM.
To further identify the protein kinase responsible for phosphorylation of the channel β-subunit, we carried out a number of kinase inhibitor studies. Only inhibitors known to affect casein kinase 2 (CK2) inhibited phosphorylation of the rod channel β-subunit (data not shown).
The expression of casein kinase 2 in ROS was investigated using specific anti-CK2 antibodies. Western blotting studies confirmed the presence of an immunoreactive CK2 variant in the soluble fraction of ROS (Fig. 7). This variant migrated with an apparent molecular mass slightly higher than that of human recombinant CK2. Immunofluorescence labeling studies further confirmed the presence of CK2 in ROS (data not shown).
The ability of the soluble extract from bovine ROS to phosphorylate bovine casein was examined. As shown in Fig. 8A, ROS soluble fraction catalyzed the phosphorylation of bovine casein.
The ability of recombinant CK2 to phosphorylate the β-subunit was also examined. Fig. 8B shows that the β-subunit and to a lesser extent the α-subunit of the rod CNG channel is phosphorylated by exogenous CK2. Together, these results suggest that the β-subunit in rod CNG channel can be phosphorylated by a CK2 variant present in ROS.
The effect of phosphorylation on the rod channel activity was determined using ion flux assays. Endogenous CK2 kinase or exogenous recombinant CK2 kinase catalyzed phosphorylation of the channel had no effect on its K1/2 or cooperativity for cGMP.
These studies indicate that the β-subunit of the rod channel can be phosphorylated by an endogenous CK2, but this posttranslational modification does not affect the sensitivity of the channel for cGMP. It is possible that phosphorylation may influence channel properties not measured by these ion flux assays or alter another function of the channel such as its interaction with other ROS proteins. More studies are required to fully assess the possible role of channel phosphorylation in ROS structure and function.
Interaction of the Rod Channel with Other ROS Membrane Proteins
In addition to CaM, the rod channel also interacts with other ROS proteins. Cross-linking and immunoprecipitation studies have revealed that the channel is associated with the Na/Ca-K exchanger in the plasma membrane.39,55,56These studies are in agreement with an earlier report suggesting an association of the channel and exchanger based analysis of ion flux measurements in vesicle preparations.57 The exchanger, which exists as a dimer in the ROS plasma membrane,58 interacts with the α-subunit of the channel.56The stoichiometry of the complex is not known, although it has been suggested that one channel interacts with two exchangers on the basis of activity measurements.56
More recently, several studies have indicated that the channel-exchanger complex also interacts with disc membrane proteins.38,39Using chemical cross-linking and immunoprecipitation approaches, we have shown that the channel associates with peripherin-2 homomeric and peripherin-2:rom1 heteromeric complexes localized along the rim region of the disc membrane.39This interaction appears to be mediated through the GARP part of the β-subunit of the channel since GARP2 also interacts with peripherin-2-containing oligomeric complexes. In contrast, the channel and soluble GARP proteins do not appear to interact with ABCR or guanylate cyclase based on immunoprecipitation and cross-linking studies.39
These studies lead to a model in which the channel associates with the exchanger in the ROS plasma membrane.39 This complex interacts with peripherin-2:rom-1 complexes to form a large multimeric complex that spans the narrow gap between the disk and plasma membrane (Fig. 9). The interaction between the channel and peripherin-2 may be responsible, at least in part, for maintaining the defined spacing between the disks and plasma membrane and for anchoring the channel:exchanger complex in the ROS plasma membrane.
Conclusions
When the rod CNG channel was first purified, cloned and expressed in heterologous cells, it was generally thought to be a relatively simple oligomeric complex consisting of a single type of subunit. Since these pioneering studies, it has become clear that the rod channel is exceedingly complex in structure, function and regulation. It consists of two subunits that assemble into a heterotetrameric complex. Each subunit confers different properties to the channel. The α-subunit is critical for cGMP-gated channel activity and for interaction with the Na/Ca-K exchanger. The β-subunit, on the other hand, contributes to the ion permeation properties of the channel, CaM-dependent modulation of the sensitivity of the channel for cGMP, and interactions with peripherin-2 complexes in the disk membrane. Thus, a picture is emerging where the channel is part of a large multiprotein complex that not only controls the flow of cations into and out of the ROS as part of the phototransduction process and Ca2+ homeostasis, but also stabilizes the unique structure of the ROS via GARP-mediated interactions with peripherin-2 complexes in the disk membranes.
Although remarkable progress has been made on characterizing the structure, function and regulation of the channel complex and its interaction with other ROS proteins, one cannot help but think that we have merely scratched the surface. The challenge for the future will be to define the structural, functional and regulatory properties of the rod channel in order to provide more detail to more fully define its role in both phototransduction and rod photoreceptor cell function and structure.
Acknowledgements
The work was supported by a grant from the National Eye Institute (EY02422) and Canadian Institutes for Health Research (MT 5822).
References
- 1.
- Burns ME, Baylor DA. Annu Rev Neurosci. 2001;24:779–805. [PubMed: 11520918]
- 2.
- Yau KW, Baylor DA. Annu Rev Neurosci. 1989;12:289–327. [PubMed: 2467600]
- 3.
- Stryer L. Annu Rev Neurosci. 1986;9:87–119. [PubMed: 2423011]
- 4.
- Pugh EN Jr, Lamb TD. Vision Res. 1990;30:1923–1948. [PubMed: 1962979]
- 5.
- Yau KW. Invest Ophthalmol Vis Sci. 1994;35:9–32. [PubMed: 7507907]
- 6.
- Yau KW, Nakatani K. Nature. 1985;313:579–82. [PubMed: 2578628]
- 7.
- Schnetkamp PP, Basu DK, Szerencsei RT. Am J Physiol. 1989;257:C153–C157. [PubMed: 2502022]
- 8.
- Pugh EN Jr, Nikonov S, Lamb TD. Curr Opin Neurobiol. 1999;9:410–418. [PubMed: 10448166]
- 9.
- He W, Cowan CW, Wensel TG. Neuron. 1998;20:95–102. [PubMed: 9459445]
- 10.
- Koch KW, Stryer L. Nature. 1988;334:64–66. [PubMed: 2455233]
- 11.
- Koutalos Y, Yau KW. Trends Neurosci. 1996;19:73–81. [PubMed: 8820871]
- 12.
- Polans A, Baehr W, Palczewski K. Trends Neurosci. 1996;19:547–54. [PubMed: 8961484]
- 13.
- Palczewski K, Polans AS, Baehr W. et al. Bioessays. 2000;22:337–50. [PubMed: 10723031]
- 14.
- Gorczyca WA, Polans AS, Surgucheva IG. et al. J Biol Chem. 1995;270:22029–22036. [PubMed: 7665624]
- 15.
- Dizhoor AM, Olshevskaya EV, Henzel WJ. et al. J Biol Chem. 1995;270:25200–25206. [PubMed: 7559656]
- 16.
- Mendez A, Burns ME, Sokal I. et al. Proc Natl Acad Sci U S A. 2001;98:9948–9953. [PMC free article: PMC55558] [PubMed: 11493703]
- 17.
- Kawamura S. Nature. 1993;362:855–857. [PubMed: 8386803]
- 18.
- Hsu YT, Molday RS. Nature. 1993;361:76–79. [PubMed: 7678445]
- 19.
- Gordon SE, Downing-Park J, Zimmerman AL. J Physiol. 1995;486:533–546. [PMC free article: PMC1156544] [PubMed: 7473217]
- 20.
- 21.
- Kaupp UB. Curr Opin Neurobiol. 1995;5:434–442. [PubMed: 7488843]
- 22.
- Zimmerman AL. Curr Opin Neurobiol. 1995;5:296–303. [PubMed: 7580151]
- 23.
- Zagotta WN, Siegelbaum SA. Annu Rev Neurosci. 1996;19:235–263. [PubMed: 8833443]
- 24.
- Biel M, Zong X, Hofmann F. Adv Second Messenger Phosphoprotein Res. 1999;33:231–250. [PubMed: 10218121]
- 25.
- Molday RS, Kaupp UB. 2000Ion Channels of Vertebrate Photoreceptors, Vol. 3, Elsevier, Amsterdam.
- 26.
- Kaupp UB, Niidome T, Tanabe T. et al. Nature. 1989;342:762–766. [PubMed: 2481236]
- 27.
- Chen TY, Peng YW, Dhallan RS. et al. Nature. 1993;362:7647. [PubMed: 7682292]
- 28.
- Korschen HG, Illing M, Seifert R. et al. Neuron. 1995;15:627–636. [PubMed: 7546742]
- 29.
- Bradley J, Frings S, Yau KW. et al. Science. 2001;294:2095–2096. [PMC free article: PMC2901924] [PubMed: 11764791]
- 30.
- Dhallan RS, Macke JP, Eddy RL. et al. J Neurosci. 1992;12:3248–3256. [PMC free article: PMC6575660] [PubMed: 1379636]
- 31.
- Chen TY, Illing M, Molday LL. et al. Proc Natl Acad Sci U S A. 1994;91:11757–11761. [PMC free article: PMC45311] [PubMed: 7526403]
- 32.
- Wohlfart P, Haase W, Molday RS. et al. J Biol Chem. 1992;267:644–648. [PubMed: 1370452]
- 33.
- Root MJ, MacKinnon R. Neuron. 1993;11:459–466. [PubMed: 7691102]
- 34.
- Eismann E, Muller F, Heinemann SH. et al. Proc Natl Acad Sci U S A. 1994;91:1109–1113. [PMC free article: PMC521463] [PubMed: 7508120]
- 35.
- Molday RS, Molday LL, Dose A. et al. J Biol Chem. 1991;266:21917–21922. [PubMed: 1718987]
- 36.
- Colville CA, Molday RS. J Biol Chem. 1996;271:32968–32974. [PubMed: 8955140]
- 37.
- Sugimoto Y, Yatsunami K, Tsujimoto M. et al. Proc Natl Acad Sci U S A. 1991;88:3116–3119. [PMC free article: PMC51396] [PubMed: 2014230]
- 38.
- Korschen HG, Beyermann M, Muller F. et al. Nature. 1999;400:761–766. [PubMed: 10466724]
- 39.
- Poetsch A, Molday LL, Molday RS. J Biol Chem. 2001;276:48009–48016. [PubMed: 11641407]
- 40.
- He Y, Ruiz M, Karpen JW. Proc Natl Acad Sci U S A. 2000;97:895–900. [PMC free article: PMC15427] [PubMed: 10639176]
- 41.
- Doyle DA, Morais Cabral J, Pfuetzner RA. et al. Science. 1998;280:69–77. [PubMed: 9525859]
- 42.
- Ruiz ML, Karpen JW. Nature. 1997;389:389–392. [PubMed: 9311781]
- 43.
- Hsu YT, Molday RS. J Biol Chem. 1994;269:29765–29770. [PubMed: 7525588]
- 44.
- 45.
- Molday RS. Curr Opin Neurobiol. 1996;6:445–452. [PubMed: 8794100]
- 46.
- Liu M, Chen TY, Ahamed B. et al. Science. 1994;266:1348–1354. [PubMed: 7526466]
- 47.
- Weitz D, Zoche M, Muller F. et al. EMBO J. 1998;17:2273–2284. [PMC free article: PMC1170571] [PubMed: 9545240]
- 48.
- Grunwald ME, Yu WP, Yu HH. et al. J Biol Chem. 1998;273:9148–9157. [PubMed: 9535905]
- 49.
- Cook NJ, Hanke W. et al. Proc Natl Acad Sci U S A. 1987;84:585–589. [PMC free article: PMC304255] [PubMed: 2432613]
- 50.
- Kuhn H, Hall SW. et al. FEBS Lett. 1984;176:473–478. [PubMed: 6436059]
- 51.
- Gordon SE, Brautigan DL, Zimmerman AL. Neuron. 1992;9:739–748. [PubMed: 1382474]
- 52.
- Molokanova E, Maddox F, Luetje CW. et al. J Neurosci. 1999;19:4786–4795. [PMC free article: PMC6782659] [PubMed: 10366613]
- 53.
- Molokanova E, Trivedi B, Savchenko A. et al. J Neurosci. 1997;17:9068–9076. [PMC free article: PMC6573590] [PubMed: 9364053]
- 54.
- Savchenko A, Kraft TW, Molokanova E. et al. Proc Natl Acad Sci U S A. 2001;98:5880–5885. [PMC free article: PMC33307] [PubMed: 11320223]
- 55.
- Molday RS, Molday LL. Vision Res. 1998;38:1315–1323. [PubMed: 9666999]
- 56.
- Schwarzer A, Schauf H, Bauer PJ. J Biol Chem. 2000;275:13448–13454. [PubMed: 10788457]
- 57.
- Bauer PJ, Drechsler M. J Physiol. 1992;451:109–131. [PMC free article: PMC1176153] [PubMed: 1328615]
- 58.
- Schwarzer A, Kim TSY, Hagen V. et al. Biochemistry. 1997;36:13667–13676. [PubMed: 9354636]
Figures
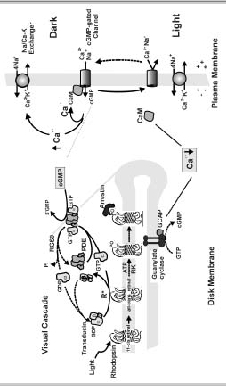
Figure 1
Basic biochemical reactions responsible for phototransduction in rod outer segments. Photoexcitation (solid lines) is initiated when light converts 11-cis-retinal to its all-trans isomer in rhodopsin. Activated rhodopsin, known as Meta II rhodopsin or R*, catalyzes the exchange of GDP bound to the α-subunit of transducin for GTP. The α-subunit dissociates from the βγ-subunit of transducin and associates with the γ-subunit of phosphodiesterase (PDE). Activated PDE catalyzes the hydrolysis of cGMP to 5'-GMP resulting in a decrease in intracellular cGMP concentration, a closure of the rod CNG channel to the influx of Na and Ca2+, and a hyperpolarization of the rod cell. The intracellular concentration of Ca2+ decreases as the Na/Ca-K exchanger continues to extrude Ca2+ from the outer segment. Recovery (dashed lines) of the ROS to its dark state occurs by the shutoff of the visual cascade and resynthesis of cGMP. Rhodopsin is inactivated through rhodopsin kinase (RK) catalyzed phosphorylation and the binding of arrestin. Transducin and PDE are returned to their inactive state by RGS9-mediated hydrolysis of GTP bound to transducin. Low Ca2+, resulting from photoexcitation, leads to the activation of guanylate cyclase via GCAP proteins. It also causes the dissociation of calmodulin (CaM) from the channel, increasing the sensitivity of the channel of cGMP. The increase in cGMP concentration leads to the reopening of the channel and an increase in intracellular Ca2+ as part of the negative feedback mechanism.
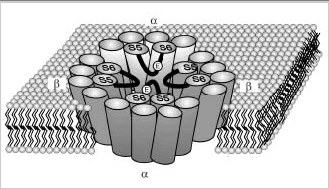
Figure 2A
Schematic diagrams of the rod CNG channel. A. Linear and topological models for the α- and β-subunits of the bovine rod CNG channel. Basic structural features include 6 transmembrane segments (S1S6), a pore region, and a cGMP binding domain. In addition, the β-subunit contains the calmodulin (CaM) binding site and the large GARP part on the N terminus. The α-subunit contains an oligosaccharide (CHO) chain on the extracellular side of the membrane and a glutamate (E) residue in the pore region that are not present on the β-subunit. GARP, in addition to being part of the β-subunit, is also present in ROS as two spliced variants referred to as GARP1 and GARP2. B. Schematic model of the rod CNG channel showing a tetrameric arrangement of two α- and two β-subunits and a central cavity. The stoichiometry of the native channel, however, remains to be determined.
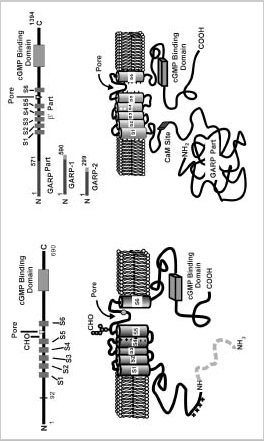
Figure 2B
See figure legend previous figure.
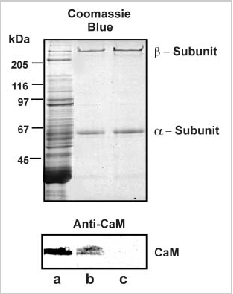
Figure 3
Copurification of CaM and the rod CNG channel from bovine ROS. ROS membranes were washed in HEPES buffer containing either 1 μM CaCl2 or 2 μM EDTA. After solubilization in CHAPS detergent, the rod CNG channel was purified on a PMc 1D1 antichannel-Sepharose matrix. The samples were subjected to SDS gel electrophoresis and the proteins were stained with Coomassie Blue (top) and Western blots were labeled with an antiCaM monoclonal antibody (bottom). Lane a: ROS; lane b: purified channel (α- and β-subunits) isolated from ROS prewashed in the presence of CaCl2; lane c: purified channel (α- and β-subunits) isolated from ROS prewashed in the presence of EDTA. Either 40 mg of ROS or 1.5 mg of purified channel were applied to the respective lanes. CaM coprecipitated with the channel from ROS washed in the presence of CaCl2, but not in the presnce of EDTA.
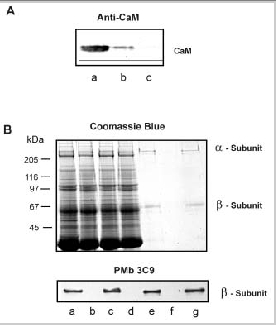
Figure 4
Interaction of the channel with CaM-Sepharose in the presence ROS soluble fraction with and without endogeneous CaM. A) Depletion of CaM from the ROS soluble fraction. ROS were lysed in hypotonic buffer containing EDTA and the soluble fraction containing CaM was separated from the membrane fraction by centrifugation. CaM was removed from the ROS soluble fraction on an CaM antibody-Sepharose affinity matrix. Western blots of ROS lysate (lane a), ROS lysate diluted 1:10 (lane b), ROS lysate depleted of calmodulin (lane c) were labeled with an antiCaM antibody. B) ROS membranes were washed in a hypotonic buffer containing 2 μM EDTA and solubilized in CHAPS. CaM-Sepharose was incubated with solubilized ROS in the presence of CaCl2 and containing either HEPES buffer (HEPES control); ROS soluble fraction containing endogenous CaM (+CaM); or ROS soluble fraction depleted of CaM (CaM). The unbound fraction from the CaM-Sepharose was separated from the bound, EDTA eluted fraction. SDS gels were stained with Coomassie Blue and Western blots were labeled for the β-subunit with the PMb 3C9 monoclonal antibody. Lane a: bovine ROS; lane b: unbound fraction (HEPES control); lane c: unbound fraction (+CaM); lane d: unbound fraction (CaM); lane e: bound fraction (HEPES control); lane f : bound fraction (+CaM); lane g: bound fraction (CaM). Endogenous CaM inhibited the binding of the channel to CaM-Sepharose.
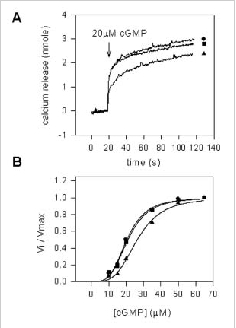
Figure 5
Bovine rod CNG channel is modulated by endogenous CaM, but not other Ca2+-binding proteins. CHAPS-solubilized ROS membrane proteins were reconstituted into Ca2+containing lipid vesicle. Potential soluble channel modulators (HEPES buffer), ROS soluble fraction containing endogenous CaM (+CaM), or ROS soluble fraction depleted of endogenous CaM (CaM) were added to the vesicles, and cGMP-dependent Ca2+ efflux was measured using the Arsenazo III assay. A. Time course and B. normalized rate as a function of cGMP concentration for cGMP-dependent Ca2+ efflux from vesicles treated with 100 ml of HEPES buffer (l), ROS soluble fraction (+CaM) (s), and ROS soluble fraction (CaM) (n). The data in B was analyzed with a Sigma Plot curve fit program of using the Hill equation V/Vmax = [cGMP]n/([cGMP]n + Kmn) where Km and n are the Michaelis constant and the Hill coefficient, respectively. The cGMP-gated channel incubated with HEPES (l) had a Km = 20.5 μM and n = 3.7, the channel incubated with a ROS lysate containing calmodulin(s) had a Km = 26.5 μM and n = 3.7 and the channel incubated with a ROS lysate depleted of calmodulin (n) had a Km = 21.1 μM and n = 3.6. These results indicate that endogenous CaM, but not other soluble proteins, modulates the activity of the channel.
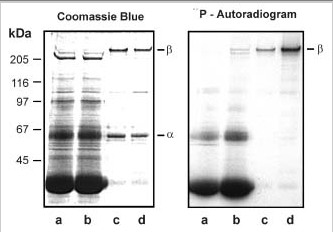
Figure 6
Phosphorylation of the rod cGMP-gated channel β-subunit by protein kinase(s) in the ROS soluble fraction. ROS membranes were hypotonically lysed, washed by centrifugation, and resuspended in either HEPES buffer or ROS lysate containing endogenous ROS soluble proteins. The phosphorylation reaction was initiated by addition of [γ-32P]ATP, and the samples were incubated for 15 min at 30°C. The phosphorylated membranes were washed in HEPES and solubilized in CHAPS detergent. The cGMP-gated channel complex that bound to the PMc 1D1-Sepharose matrix was separated from the unbound fraction. Proteins were resolved on a 9% SDS-polyacrylamide gel and stained with Coomassie Blue and subjected to autoradiography for analysis of phosphorylated proteins. lane a, unbound fraction for ROS membranes phosphorylated in HEPES; lane b, unbound fraction for ROS phosphorylated in the presence of ROS soluble fraction; lane c, bound fraction containing the channel (α- and β-subunits) for ROS phosphorylated in HEPES; lane d, bound fraction containing the channel (α- and β-subunits) for ROS phosphorylated in the presence of ROS soluble fraction. The β-subunit is most intensely labeled when phosphorylation is carried out in the presence of ROS soluble fraction.
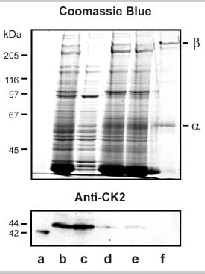
Figure 7
Soluble fraction of ROS contain a CK2 α-subunit variant. Human recombinant casein kinase II (lane a), bovine ROS (lane b), ROS cytosol (lane c), solubilized ROS membranes (lane d), unbound (lane e) and bound (lane f) fraction from PMc 1D1-Sepharose were subjected to SDS gel electrophoresis and either stained with Coomassie Blue or transferred to Immobilon-P membranes and labeled with an anti-CK2a monoclonal antibody. Lanes contained either 40 mg of ROS or 3 mg of purified channel. CK2 α-subunit is present in the soluble fraction from hypotonically lysed ROS and migrates with a slightly higher apparent molecular weight relative to recombinant CK2 α.
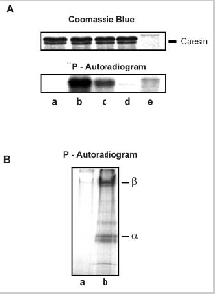
Figure 8
Phosphorylation of caesin by ROS soluble fraction and the cGMP-gated channel by a human recombinant CK2 A. The ability of a soluble fraction from ROS to phosphorylate bovine milk casein was examined by autoradiography. lane a: casein alone; lane b: casein phosphorylated using a hypotonic soluble fraction from ROS; lane c: casein phosphorylated using a diluted (1:30) ROS soluble fraction; lane d: casein phosphorylated using ROS lysate depleted of CK2 by immunoprecipitation; lane e: phosphorylated ROS lysate. B. Phosphorylation of the rod channel by recombinant CK2. Washed ROS membranes were solubilized in CHAPS and the cGMP-gated channel bound to PMc 1D1-Sepharose. The bound channel complex was phosphorylated in the presence of CHAPS detergent by the addition of exogenous CK2 (2.3 U), eluted from the column and subjected to SDS gel electrophoresis. Phosphorylation of the channel was detected by autoradiography. lane a: purified channel incubated with [γ-32P]ATP; lane b: purified channel incubated with exogenous CK2 and [γ-32P]ATP.
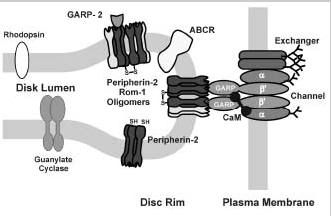
Figure 9
Diagram depicting the interaction of the rod channel with other ROS proteins as revealed by immunoprecipitation and cross-linking studies. In the plasma membrane, the heterotetrameric cGMP-gated channel (depicted as consisting of two α-subunits and two β-subunits) interacts with Na/Ca-K exchanger39,55,56 previously shown to exist as a dimer in the membrane.58 The β-subunit of the channel binds endogenous CaM in a Ca2+-dependent manner as shown in this study. The channel:exchanger complex also interacts with peripherin-2-containing oligomers in the rim region of disc membranes. This interaction is mediated by the GARP part of channel β-subunit. GARP2 and less abundant GARP1 (not shown) are confined to the rim region of disc membranes through their interactions with peripherin-2 homomeric and peripherin-2:rom1 heteromeric disulfide-linked oligomeric and core complexes.38,39 Disc proteins ABCR and GC1 (guanylate cyclase) do not form stable associations with the cyclic GMP-gated channel or soluble GARP proteins.